The authors would like to acknowledge James (Ned) Williams for his work on the previous edition. Injury to tissue initiates a complex series of events involving many cellular and biochemical responses that ultimately result in wound healing. The series of events depends on the severity of the injury and the tissues involved. The goal is regeneration or repair of the injured or traumatized tissue. Bone generally restores tissue architecture by regeneration of the normal cell population while other connective tissues respond by repair of the injured area.1 The wound repair response is generally described by three major phases: inflammation, reparative (proliferative or fibroblastic), and remodeling (maturation).1–3 These phases of healing overlap, and their sequence and timing vary with the tissue involved and the severity of the injury. The focus of this chapter is the basic wound healing response as well as the specific healing patterns of bone, muscle, tendon, ligament, and cartilage. Most tissues undergo an initial inflammatory phase that involves an acute vascular response followed by cellular infiltration.1,3 The immediate vascular component of the response is centered around hemostasis in the wound. Blood vessel disruption within the wound allows extravascular movement of blood elements and exposure of subendothelial collagen to platelets, causing activation of the coagulation cascade.1,3–5 The result is formation of a fibrin network to support the hemostatic plug and act as a scaffold for cellular infiltration. The cellular aspect of the inflammatory phase follows the initial vascular response. The release of cytokines associated with the hemostatic plug and increased vascular permeability incites the chemotaxis of cells into the wound environment (Figure 6-1). Neutrophils are the first cells migrating into the area, appearing by 6 hours after injury and peaking over a 2- to 3-day period.1,3,5 The roles of the neutrophil are initial debridement and phagocytosis of microorganisms, thereby minimizing the potential for infection. The extent of neutrophil action within the wound depends on the severity of the wound and degree of contamination. The contribution by neutrophils in a noncontaminated wound is not essential for normal wound healing.1,5 Macrophages appear within the wound approximately 24 to 48 hours after neutrophil migration.1 The influx of macrophages into the wound appears key to the transition from the inflammatory phase to the reparative phase and appropriate wound repair.1,3 They are central to five major functions: phagocytosis, wound debridement, matrix synthesis regulation, cell recruitment and activation, and angiogenesis.3 Phagocytosis and wound debridement occur with the release of oxygen radicals, nitric oxide, and collagenases.3 Working in conjunction with neutrophils, macrophages create an optimal environment for entering the reparative (fibroblastic) phase of wound healing. This activity is at a maximum in the first 3 to 4 days after injury. In addition, macrophages release a host of cytokines, growth factors, prostaglandins, and enzymes that subsequently activate and mediate angiogenesis and fibroplasia.3 The macrophage appears to be the central figure in control of cellular and biochemical events in general wound healing. The presence of macrophages also attracts and activates lymphocytes, whose function remains controversial.1,3 Lymphocytes secrete lymphokines such as interferons and interleukins that stimulate fibroblast migration and collagen synthesis.1,3,6 Their activity appears to peak approximately 6 days after injury.1,5 The reparative (proliferative or fibroblastic) phase of wound healing is characterized by the cellular response of endothelial cells and fibroblasts.1,3 Fibroblast proliferation and migration predominate and are followed by matrix synthesis, including collagen, elastin, and proteoglycans. The fibroblasts begin appearing in the wound within 3 days of injury; however, there is a lag phase of 2 to 3 days before initiation of collagen production with maximal proteoglycan synthesis not occurring until 2 weeks after injury.5 Matrix synthesis increases over the following few weeks with concurrent increases in tensile strength.1 In addition, endothelial cells adjacent to the wound begin to proliferate and form new capillaries that gradually migrate into the wound. The newly developing capillaries follow immediately behind the migrating fibroblast and collagen scaffold and continue until normal oxygen tension in the wound is restored.5 This dense network of macrophages, fibroblasts, and neovascularization during the proliferative phase is generally recognized as granulation tissue. The remodeling phase is the final aspect of wound healing during which collagen fibers reorient parallel to lines of stress and strain and the fibers cross link in a stable formation.7,8 This is the most important aspect of wound healing for connective tissues, because appropriate collagen deposition and alignment are critical to adequate tensile strength development in the repaired tissue. Although collagen deposition reaches a maximal point 2 to 3 weeks after injury, tensile strength continues to progressively increase over the course of approximately 1 year (Box 6-1 and Box 6-2). This period allows for removal of biomechanically inferior collagen fibers (type III) and replacement with the fibers suitable for the specific tissue involved (generally type I collagen). In addition, decreasing proteoglycan concentration leads to a decrease in water content, resulting in compression of the collagen fibers. As fibers become closer in proximity because of realignment and compression, increased surface area is available for cross-linking, subsequently increasing tensile strength.5 Thus a proper equilibrium between collagenolysis and matrix accumulation becomes essential. Bone is a dynamic tissue composed of 35% organic material and 65% mineral.2,9–11 The organic portion comprises cellular constituents (osteocytes, osteoblasts, and osteoclasts) and matrix components. The osteocytes are cells of mature bone that remain within spaces (lacunae) surrounded by lamellar bone and are responsible for normal mineral homeostasis. Osteoblasts are responsible for osteoid production, and osteoclasts function in bone resorption.11,12 A fine balance of bone resorption and deposition is maintained for mineral homeostasis as well as continuous remodeling secondary to mechanical stresses placed on the bone in accordance with Wolff’s law which states that a bone in a healthy individual will adapt to the forces under which it is placed.12,13 Bone matrix is composed of predominately type I collagen and ground substance containing glycosaminoglycans.8,11,12 Calcium and phosphorus deposit in association with the collagen fibrils and serve as the primary reservoir for overall calcium homeostasis. Hydroxyapatite crystals of calcium and phosphorus are aligned with the collagen fibrils and provide the structural rigidity to bone.11,12 This unique arrangement between collagen and mineral creates the viscoelastic property of bone with the crystal supplying resistance to compressive forces and the collagen providing tensile support.12 Blood supply to bone is primarily based on the nutrient artery that bifurcates into ascending and descending branches within the medullary cavity (Figure 6-2). In addition, there is vascular support from metaphyseal arteries and periosteal arterioles.11 The metaphyseal vessels anastomose with the nutrient artery branches within the medullary canal to provide complete collateral circulation. The vascular flow is centrifugal, with elevated pressure in the medullary cavity driving blood toward the periosteum. The efferent system is a venous drainage system using periosteal venules and the medullary venous sinus. Cortical vessels flow through Haversian canals in which small vascular channels are surrounded by layers of lamellar bone.9–11 These channels in the cortical bone provide perfusion to the osteocytes within their lacunae.12 The periosteal arterioles are the least important contributors of the vascular supply in healthy intact bone and are primarily of concern in areas of soft tissue attachment. Injury to the bone results in disruption of the vascular supply to varying degrees depending on the severity of the fracture. If the endosteal supply remains intact, it will predominate during healing. However, if the endosteal supply is disrupted, as in most fractures, the periosteal support becomes extremely important, thus making careful maintenance and handling of soft tissue attachments crucial to healing (Box 6-3).14 As discussed previously, bone is one of the few tissues that can undergo direct cellular regeneration to restore 100% of the original biomechanical properties. This can occur through two major types of healing, primary or secondary. Primary, or direct, bone healing occurs when there is a minimal fracture gap with rigid stability, and it proceeds by either contact or gap healing. Contact healing involves the direct formation of bone across a fracture line less than 0.1 mm wide by creating resorption cavities, or “cutting cones,” through the Haversian canals parallel to the long axis of the bone (Figure 6-3).9,10,15,16 Osteoclasts lead the front edge of the resorption cavity across the fracture line and remove bone and calcified matrix in preparation for osteoblastic activity immediately following. Finally, nutritional support is supplied by capillary loops following the advancing osteoblasts.9,15,16 This progressive Haversian remodeling continues across the fracture line until the surrounding bone has been remodeled into new Haversian systems. When the fracture line is greater than 0.1 mm but less than 0.5 mm, primary bone union can still occur through gap healing.10,16 This distance cannot be directly crossed by a resorption cavity. As a result, lamellar bone produced from cells lining the medullary cavity and periosteum first fills the void in a transverse direction. Subsequently, resorption cavities cross the newly formed lamellar bone and again create new Haversian systems in the proper longitudinal direction.10,16 In fracture repair, both gap and contact healing may occur in different portions of the same fracture line.16 With primary bone healing, there is little radiographic callus formation, and the fracture line gradually disappears. Although direct healing entails direct formation of lamellar bone and remodeling of Haversian systems without intermediate fibrous or cartilaginous tissue, it does not reduce the time for fracture healing, and weeks to months may still be required before complete union.15 In fact, early stability is generally decreased when compared with secondary healing, typically requiring longer periods before removal of stabilizing implants.17 Radiographic evaluation is generally performed at 4- to 6-week intervals until the fracture line has resolved. The goal is to achieve recovery of function and early weight-bearing with implants employing interfragmentary compression so rehabilitation can begin at an early stage. Secondary, or indirect, bone healing occurs when there is less rigidity or absence of anatomic reduction such that the fracture gap is greater than 0.5 mm and is characterized by callus formation (see Figure 6-3).16,18 The degree of callus formation is related to the amount of instability at the fracture site. In general, secondary bone healing proceeds through the same phases as basic wound healing.9,10,19 Similar to basic wound healing, hemostasis is the initial response at the time of injury, initiating the inflammatory phase. Immediate hemorrhage and hematoma formation occur within the fracture gap in addition to the surrounding soft tissues that may be damaged. Destruction of the Haversian canals at the site of injury causes osteocyte death and necrosis of bone. The process proceeds with the migration of neutrophils followed by macrophages into the injured site for removal of necrotic debris. In addition, biochemical mediators such as bone morphogenetic proteins, platelet-derived growth factor, osteonectin, prostaglandins, and osteocalcin enter the fracture site to contribute to bone healing.10 As the inflammatory response previously described subsides (24 to 72 hours), the repair process begins.9,10,19 The reparative phase begins with the surrounding tissue providing mesenchymal cells, which enter the fracture site to form osteoblasts, chondroblasts, and fibroblasts. During the initial 3 weeks, a fibrous and cartilaginous “soft” callus is formed by the deposition of types I, II, III, V, IX, and X collagen synthesized by mesenchymal cells. Types III and V collagen initially appear during the inflammatory phase, whereas types II and IX collagen peak during the cartilaginous aspect of the reparative phase 1 to 2 weeks after injury. Collagen type I is initially present at low levels in the first 2 weeks but becomes the predominant type by day 14.8,13 The function of the soft callus is to unite the fracture ends and decrease interfragmentary motion and strain.16 The fibrocartilage deposited is gradually transformed to bone by the process of endochondral ossification similar to that which occurs during physeal growth.8,19 Chondrocytes progress to a hypertrophied stage followed by mineralization of the surrounding matrix. Invasion by capillaries brings cells that resorb the mineralized cartilage matrix, produce osteoid, and deposit bone. This transition from soft cartilaginous callus to hard bony callus is directly related to the blood supply available and the interfragmentary strain at the fracture gap. With high interfragmentary strain or poor blood supply, activity of osteoblasts and osteoid production is limited, and the callus remains as fibrocartilage.10,16 The remodeling or maturation phase proceeds following the transition from soft to hard callus. The fracture gap is bridged and stabilized with endosteal and periosteal callus consisting primarily of woven bone that must be remodeled to lamellar bone according to weight-bearing forces as directed by Wolff’s law. In addition, the normal blood supply is reestablished. As the endosteal callus is gradually remodeled, the medullary cavity and its blood supply are restored. Similarly, as cortical bone is restored, the Haversian system is re-created and the normal centrifugal vascular pattern can return with concurrent resorption of the temporary extraosseous supply.18,19 Biomechanical factors at the fracture site are important to healing. Wolff’s law has a tremendous effect on bone deposition and resorption. The stress placed on the bone stimulates healing as long as the micromotion created does not exceed the acceptable interfragmentary strain for osteoid deposition.18 If the mechanical load exceeds the strength of the reparative tissues, inhibition of bone healing or re-fracture occurs. Thus it is important that no or minimal motion between fracture fragments occurs early in the healing phase to allow adequate establishment of the extraosseous blood supply. After the vascular supply is established and callus formation has occurred, then controlled axial micromotion can stimulate appropriate bone remodeling. As such, destabilization of external fixator frames has been recommended at approximately 4 to 6 weeks following frame application to “dynamize” the fracture line.20,21 Electrical fields have been demonstrated in normal and injured bone and are related to either mechanical forces or bioelectric potentials.22 Strain-related potentials occur secondary to mechanical loading of the bone and are related to piezoelectric activity resulting from collagen deformation and fluid flowing through space in the extracellular matrix.10 Bioelectric potentials are generated by cellular functions associated with metabolic processes in the cell.22 These electrical fields can be stimulated exogenously and have been demonstrated to favorably affect bone remodeling. Electrical stimulation can be provided by invasive or noninvasive methods. Invasive stimulation includes direct surgical implantation of a cathode at the site of stimulation and the anode placed in the surrounding soft tissue. Noninvasive stimulation can be accomplished by capacitive or inductive coupling of current to the appropriate site. With capacitive coupling, the electrodes are placed on the skin on opposite sides of the stimulation site, and electrical fields between 1 and 10 mV/cm are generated. Inductive coupling uses external electromagnetic coils to establish an electromagnetic field of time-varying or pulsed nature to create a secondary electrical field in the bone.22 Currently, the mechanism of electrical stimulation of bone healing is not completely understood, but its use has been advocated with nonunion fractures.23,24 Alterations in endocrine balance can influence bone healing as well. Hormones such as parathormone, calcitonin, vitamin D, and thyroid may affect bone resorption and deposition.10 Corticosteroids and diabetes mellitus can have inhibitory effects on bone healing.10,25 In addition, there are numerous other miscellaneous factors that must be considered with respect to fracture healing, including patient age, fracture configuration, fracture location, and whether the site was grafted.10,16 Young patients heal faster than geriatric individuals. The fracture configuration is also important because highly comminuted fractures or those with significant bone loss require more time to achieve bony union. Furthermore, fractures closer to the metaphysis in locations of high cancellous bone, or those that have been grafted, may have more rapid healing potential. Efforts to enhance fracture healing continue along many pathways. The ability to stimulate bone healing with the use of growth factors is a future possibility, and research continues in the field of electrical stimulation. In addition, research has demonstrated potential advantages to using ultrasound and extracorporeal shockwave technology to enhance fracture healing.24,26 Skeletal muscle is composed of aggregations of muscle cells termed fibers (myofibers), consisting of multiple nuclei within each fiber (Figure 6-4). Each individual muscle belly is composed of numerous fibers bound together by connective tissue into multiple diffuse bundles (fascicles). A dense fibrous connective tissue sheath termed the epimysium surrounds the entire muscle. The perimysium is the connective tissue support surrounding each fiber bundle within the muscle belly and the endomysium represents sheets of connective tissue surrounding each independent muscle fiber.27,28 Capillaries, nerves, and lymphatics traverse to the muscle fibers through the surrounding connective tissue sheaths, eventually reaching the endomysium, where a rich capillary network highly sensitive to sympathetic vasomotor tone is established around the individual myofibers.29
Tissue Healing
Tendons, Ligaments, Bone, Muscles, and Cartilage
General Wound Healing
Inflammatory Phase
Reparative Phase
Remodeling (Maturation) Phase
Bone Healing
Bone Structure
Primary Bone Healing
Figure 6-3 Bone healing. A, Primary bone healing with the formation of cutting cones. B, Secondary bone healing.
Secondary Bone Healing
Factors Affecting Healing
Muscle Healing
Muscle Structure
< div class='tao-gold-member'>
Stay updated, free articles. Join our Telegram channel
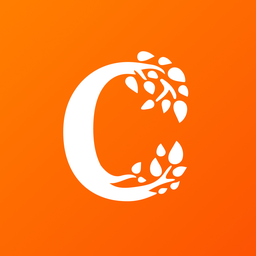
Full access? Get Clinical Tree
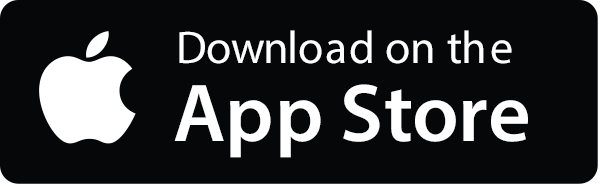
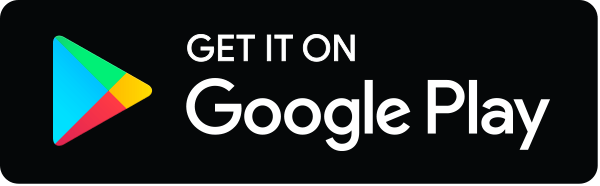