Chapter 6 Electrophysiology and arrhythmogenesis
RECORDING ELECTRICAL EVENTS IN CELLS
The depolarization process can be detected at different levels by intracellular electrodes, intracardiac electrodes and by electrodes placed on the body surface.1,2
Intracellular electrodes have been used to demonstrate the potential difference across the cell membrane, and to record the change in this potential difference which occurs during depolarization and repolarization.1 Using this method, it is possible to detect differences in transmembrane potentials in specialized tissues, to understand the differences and similarities in the electrophysiological properties of different tissues. Furthermore, it is possible to predict the changes in cell depolarization and repolarization which may result from altered electrolyte levels, drugs and, to some extent, myocardial disease.1
A method to obtain an approximation of the transmembrane voltage in vivo is to record the monophasic action potential (MAP)3 (Fig. 6.1). Using a catheter with special electrodes at the tip, introduced transvenously into right atrium or right ventricle, the MAP can be recorded when the catheter tip makes close contact with the myocardium. The orientation of the catheter tip relative to the myocardium is important when interpreting the morphology of MAP recordings.3 Therefore, it has been suggested that results of contact MAP recordings should only be used to determine action potential duration (APD).4 Determination of the APD is a useful parameter to study the effect of drugs on cardiac electrophysiological characteristics.
Intracardiac electrodes have also been used to detect the depolarization and repolarization of each tissue in the conduction network and therefore to determine the timing and duration of the activation process. This technique was used to demonstrate the different depolarization processes in different species.5 Intracardiac electrodes, introduced transvenously, provide a relatively easy way to record atrial and ventricular electrograms in horses (see Chapter 14).
The surface electrocardiogram (ECG) records the potential difference between selected points on the body surface. It allows us to detect changes in the electrical field which is built up around the heart during depolarization and repolarization. Although the body surface ECG depends on the sum of electrical forces and their conduction to the skin, it does allow clinicians to determine the heart rhythm and, with experience built up over many years of studies, to identify certain disease processes that are associated with specific ECG changes.6
CELLULAR PHYSIOLOGY
All myocytes possess the properties of excitability, refractoriness and conductivity.6 Like nervous tissue, individual cardiac cells exhibit the all-or-none phenomenon – that is once threshold is reached they are completely activated by an action potential. The cells can therefore be described as excitable. Once the action potential is initiated, cells cannot be depolarized again until they have returned to the resting potential following repolarization. This property of refractoriness ensures that all cardiac cells have a period after activation during which no level of further stimulus will cause an action potential. This prevents heart muscle developing a tetanic spasm, which would prevent relaxation and filling of the chambers, and helps to maintain an organized pattern of depolarization. Myocytes also possess the ability to conduct a stimulus for depolarization to their neighbours. Atrial and ventricular myocytes form two syncytia within which excitation passes from cell to cell through intercalated discs. Other tissues within the heart have specialized conductivity properties so that they conduct the impulse along a network either slowly (atrioventricular node) or rapidly (e.g. Purkinje fibres). While the atrial and ventricular myocytes have contractile function, the cells of the specialized conducting network have no contractile protein.
The electrophysiological features of myocytes result from specific properties of the cardiac cell membrane.1 Like all living cells, the inside of cardiac cells has a negative electrical charge compared to the outside of cells due to an accumulation of negatively charged ions. This voltage difference across the cell membrane is called the transmembrane potential, which is about −80 to −90 mV. All cells have a very high intracellular concentration of potassium and low levels of sodium and calcium. For most cells this situation remains unaltered throughout their lives. However, excitable cells like cardiac cells have tiny pores or channels in the cell membrane. Upon appropriate stimulation, these channels open and close in a predefined way to allow specific ions to move across the cell membrane. This movement of ions results in changes in the transmembrane potential, from −90 mV to about +20 mV (depolarization), and finally back to −90 mV (repolarization). A graphical presentation of these changes in transmembrane potential is known as the action potential (Fig. 6.2). The currents, and thus the morphology of the action potential, vary in different tissues and determine the different properties of each specialized cell. Also, changes in extracellular ion concentrations, disease and drugs may influence the action potential of the cells.1
The action potential can be divided into five phases, 0–41,6 (see Fig. 6.2). However, it is more practical to consider the action potential in terms of three general phases: depolarization, repolarization and resting phase.
Depolarization
Phase 0 represents the depolarization process, and begins once a cell has reached a certain potential (the threshold potential), spontaneously in the case of pacemaker cells or as a result of depolarization of adjacent cells in the case of conduction pathway and myocardial cells. The upstroke of the cardiac action potential in atrial and ventricular muscle and His-Purkinje fibres is due to a sudden increase in membrane conductance to Na+, resulting in a massive Na+ influx into the cell. The rate at which the depolarization occurs (slope of phase 0) is a determinant of the conduction velocity for the propagated action potential. In the slower conducting pacemaker tissues of the sinoatrial (SA) and atrioventricular (AV) nodes, the resting potential of the cell membrane is less negative (around –60 mV) than in nonpacemaker cells. This results in a decreased rate of phase 0 depolarization and relatively slow conduction in pacemaker tissues.1 In these cells, the contribution of the fast inward current usually carried by sodium is small, and the relatively slow depolarization process is largely due to movement of calcium ions. Consequently, these tissues are relatively sensitive to changes in calcium concentration.1
Repolarization
This phase is immediately followed by the plateau phase (phase 2) which may last several hundred of milliseconds. During this period, membrane conductance to all ions falls to rather low values, with a complex interaction of ion movements involving sodium, potassium, magnesium and chloride, and in particular an inward L-type calcium current. These channels, especially the latter, interrupt the repolarization process and prolong the action potential. As such, the action potential duration (APD), and therefore the refractory period, is predominantly determined by the balance between the inward and outward currents during the plateau phase, which have a very important role in the generation of some arrhythmias.7–9
After the plateau phase, the final rapid repolarization (phase 3) takes place due to a series of potassium currents out of the cell. In order to maintain the concentration gradients, sodium is pumped out of the cell in exchange for potassium, resulting in a return of the membrane potential towards the resting level (phase 4).1,6 During this final recovery process, the cardiac myocyte gradually regains excitability. This means that the cell is not excitable during phase 1, phase 2 and the beginning of phase 3, regardless of the magnitude of the stimulating impulse. This period is called the absolute refractory period (ARP). As the cell repolarizes, it once again becomes excitable. However, there is a period of time during which the cell can only be excited by a large current. This period is known as the relatively refractory period (RRP).10
Resting phase or diastolic depolarization
Under normal conditions, membrane potential of atrial and ventricular muscle cells remains steady at around −90 mV throughout diastole (phase 4). However, in certain specialized conducting cells or due to leakage of ions across the cell membrane, the resting membrane potential does not remain constant in diastole but gradually depolarizes. This spontaneous diastolic depolarization may reach threshold potential (around −60 mV) by itself and produce an action potential, a characteristic called automaticity.6 The rate of this change in potential is mainly determined by a time-related change in membrane potassium or sodium permeability. It is influenced by autonomic tone, electrolytes, drugs and disease. The steeper the slope of phase 4, the faster the rate of automaticity. Phase 4 is steepest in SA nodal cells. However, the AV node and junctional tissue also have automaticity and will take over as pacemaker if the SA node fails to depolarize. In some disease states, Purkinje tissue may also act as a pacemaker, and abnormal automaticity may occur in other cells.7,8 The action potentials of a ventricular and pacemaker cell, showing phases 0–4, are shown in Figure 6.2. Compared to the action potential of a ventricular or Purkinje fibre cell, the sinoatrial and atrioventricular nodal cells have a slow depolarization phase (phase 0). This slower depolarization phase occurs because of a lack of rapid sodium channels responsible for the rapid depolarization phase. The sinoatrial and atrioventricular nodes are mainly dependent on the slow calcium channel for depolarization. Because of the slower rate of depolarization, the sinoatrial and atrioventricular nodes conduct electrical pulses slowly. For the atrioventricular node this slow conduction is reflected on the surface ECG as the PR interval.
Innervation of the heart
The heart is innervated by the sympathetic and the parasympathetic nervous system. Parasympathetic fibres are carried in the vagus nerve and their discharge results in a depressed automaticity (slower heart rate), decreased conduction velocity and increased refractory period. This effect is mediated by the release of acetylcholine at nerve endings, which affects the membrane potential of pacemaker cells,6 particularly in the SA node and, in horses, in the AV node. The sympathetic nervous system acts on the heart via the release of adrenaline and noradrenaline. This results in an increased automaticity, an increased conduction velocity, a shortened refractory period (cells recover more quickly and permit a higher rate of stimulation) and an increased myocardial contractility. The subcellular effect of these hormones is largely due to effects on contractile proteins; however, they also affect transmembrane potentials and alter cardiac rhythm in some circumstances.8,11
The conduction process
The conduction process follows a predictable pathway in the normal heart, leading to a coordinated contraction of atrial and then ventricular muscle (Fig. 6.3). An impulse spreads from the SA node, across the atria, to the AV node. Conduction occurs along specialized fibres (internodal tracts); however, the impulse also leads to contraction of atrial muscle. The electrical activity associated with depolarization of this muscle mass results in a sufficiently large electrical field for it to be detected on a body surface ECG as a P wave (see Fig. 6.3A). The precise location of impulse formation within the SA node and the pattern of depolarization across the atria can be influenced by heart rate and autonomic tone, which can result in a different configuration of P waves (wandering pacemaker) even though the SA node remains the source of the impulse.12
When the impulse reaches the AV junction it finds a barrier to further spread. The specialized cells of the AV node conduct the impulse slowly. Because only a small number of cells are depolarized, no deflection is seen on the surface ECG. This period is represented by the P–R interval (see Fig. 6.3B). Conduction through the AV node is profoundly affected by vagal tone in the horse. Even in normal animals, conduction is often sufficiently slowed or reduced in amplitude to result in a marked reduction in the normal rate of conduction (first-degree AV block), or complete abolition of further spread of the impulse (second-degree AV block).13 ( AR, EA)
When the impulse passes through the AV node it is rapidly conducted through the bundle of His and the Purkinje network to the ventricular myocardium. Depolarization of the ventricles is rapid and results in a coordinated contraction. Depolarization of the Purkinje network is not detected on the body surface ECG; however, depolarization of the myocardium results in substantial electrical forces, the net result of which produce the QRS complex on the surface ECG (Fig. 6.3C).
Each cell within the heart repolarizes after depolarization. The sum of the repolarization processes within the heart can be detected at the body surface in the same way as the electromotive forces of depolarization. Ventricular repolarization is seen as the T wave. The change in electrical field caused by atrial repolarization (atrial T wave or Ta wave) may or may not be seen.6
In conclusion, deflections on the surface ECG are caused by atrial or ventricular myocardial depolarization. Depolarization of the sinus node or atrioventricular node does not result in any deflection. The slow conduction through the atrioventricular node produces the (flat) PR interval. Identification of the characteristic waveforms allows a clinician to detect when depolarization and repolarization of the atria and ventricles has occurred. The timing of the waves, the relation between the different waves, and the morphology and duration of the complexes and intervals allows deduction of the origin and conduction pathway of the impulse (see Fig. 6.3).
The cardiac electrical field
During the depolarization and repolarization processes, different currents are flowing across the cell membrane at various points, and a potential difference will be present between one part of the cell and another. When current is flowing, an external electrical field is set up around the cell, which can be described as acting as a dipole.6 However, when the cell is depolarized, or repolarized at a resting potential, no electrical field is formed around the cell because no current is flowing, despite the potential difference between the inside and outside of the cell. Each myocyte forms its own electrical field, but the electrical effects summate to produce an electrical field around the whole heart which, in simplified terms, can then be regarded as a single dipole.6
RECORDING OF CARDIAC ELECTRICAL ACTIVITY
The surface ECG
The changes in the electrical field around the heart can be detected by a galvanometer attached to the body surface, which records the potential difference between two electrodes. The link between a positive and negative electrode is called a bipolar lead. An ECG records the potential difference between electrodes placed at various points on the body surface, which reflects the sum of all the electrical fields which are present at any one time. The points at which the ECG electrodes are placed are chosen to represent electrical changes in the heart; however, a number of other factors also affect the potential difference between different areas of the body. The position of the heart within the body, the course of the spread of activation within the heart, the shape of the thorax, the conductivity of tissues between the heart and the electrodes, and the exact location of the body surface electrodes all affect the body surface ECG.12,13
The concept of cardiac vector
The surface ECG reflects the combined effects of all the electrical activity of the heart. The sum of the electromotive forces has a direction and magnitude, which is termed the cardiac vector. The ECG voltmeter will have a positive deflection if the net direction of overall activity (vector) is towards the positive electrode of a bipolar lead, and a negative potential if it is away from it (Fig. 6.4). The voltage recorded will be largest when the vector is directly towards the positive electrode. If the direction of the maximum potential difference is at an angle to the lead axis, the deflection will be smaller. If the electrodes are positioned perpendicular to the vector of electromotive force, no potential difference will be detected. The amplitude of the deflection indicates the magnitude of the vector and is proportional to the mass of myocardial tissue which is depolarized.
< div class='tao-gold-member'>
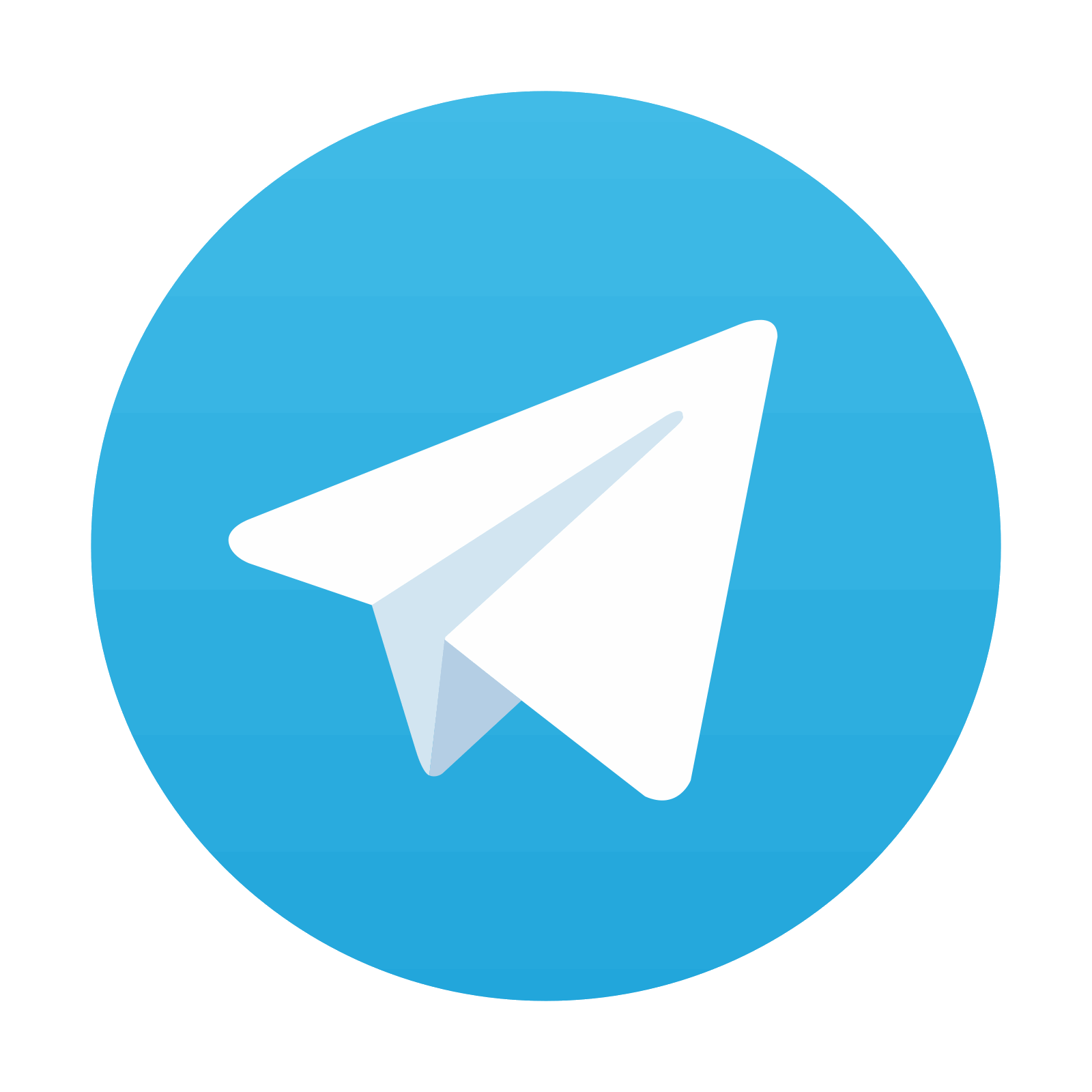
Stay updated, free articles. Join our Telegram channel
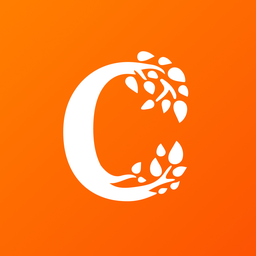
Full access? Get Clinical Tree
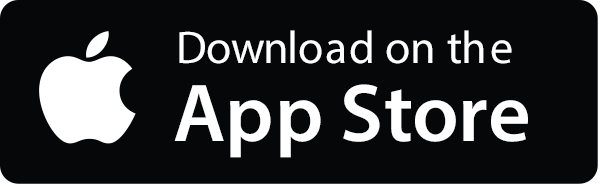
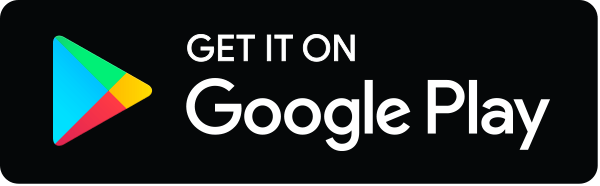