Electrical Activity of the Heart
1. Contraction of cardiac muscle cells is triggered by an electrical action potential.
2. The contractile machinery in cardiac muscle is similar to that in skeletal muscle.
3. Cardiac muscle forms a functional syncytium.
4. Cardiac contractions are initiated by action potentials that arise spontaneously in specialized pacemaker cells.
5. A system of specialized cardiac muscle cells initiates and organizes each heartbeat.
6. Cardiac action potentials are extremely long.
7. Membrane calcium channels play a special role in cardiac muscle.
8. The long duration of the cardiac action potential guarantees a period of relaxation (and refilling) between heartbeats.
9. Atrial cells have shorter action potentials than ventricular cells.
10. Specialized ion channels cause cardiac pacemaker cells to depolarize to threshold and form action potentials.
11. Sympathetic and parasympathetic nerves act on cardiac pacemaker cells to increase or decrease the heart rate.
12. Cells of the atrioventricular node act as auxiliary pacemakers and protect the ventricles from beating too fast.
13. Sympathetic nerves act on all cardiac muscle cells to cause quicker, more forceful contractions.
14. Parasympathetic effects are opposite to those of sympathetic activation but are generally restricted to the sinoatrial node, atrioventricular node, and atria.
15. Dysfunction in the specialized conducting system leads to abnormalities in cardiac rhythm (arrhythmias).
16. Atrioventricular node block is a common cause of cardiac arrhythmias.
17. Cardiac tachyarrhythmias result either from abnormal action potential formation (by the sinoatrial node or ectopic pacemakers) or from abnormal action potential conduction (“reentry”).
18. Common antiarrhythmic drugs affect the ion channels responsible for the cardiac action potential.
Contraction of Cardiac Muscle Cells Is Triggered by an Electrical Action Potential
The heart is a muscular pump that propels blood through the blood vessels by alternately relaxing and contracting. As the heart muscle relaxes, the atria and ventricles fill with venous blood. During cardiac contraction, some of this blood is ejected into the arteries. Cardiac contraction takes place in two stages: (1) the right and left atria begin to contract, and (2) after a delay of 50 to 150 milliseconds (msec), the right and left ventricles begin to contract. Atrial contraction helps to finish filling the ventricles with blood. The delay allows time for this “topping up” of ventricular volume. Ventricular contraction ejects blood out of the left ventricle into the aorta and out of the right ventricle into the pulmonary artery. After the atria and ventricles contract, they relax and begin to refill. The entire contractile sequence is initiated and organized by an electrical signal, an action potential, which propagates from muscle cell to muscle cell, through the heart.
This chapter begins with a brief description of how cardiac muscle contracts, followed by a detailed description of the action potentials that initiate and organize the heart’s contractions. Several common electrical dysfunctions of the heart are then discussed.
Throughout this chapter, comparisons are made between cardiac and skeletal muscle (Table 19-1). In both cardiac and skeletal muscle, an electrical action potential in each muscle cell is necessary to trigger a contraction. The molecular mechanisms that carry out the contraction are also similar in both types of muscle. However, important differences exist between cardiac and skeletal muscle in the characteristics of the action potentials that initiate contractions.
TABLE 19-1
Sequence of Events in Contraction of Skeletal Muscle and Cardiac Muscle
Skeletal Muscle | Cardiac Muscle |
Action potential is generated in somatic motor neuron | Note: Action potentials in autonomic motor neurons are not needed to initiate heartbeats |
Acetylcholine is released | Note: Neurotransmitters are not needed to make the heart beat |
Nicotinic cholinergic receptors on muscle cell membrane are activated | Note: Activation of receptors is not needed—a completely isolated or denervated heart still beats |
Ligand-gated Na+ channels in muscle membrane open | Pacemaker Na+ channels spontaneously open (and K+ channels close) in membranes of pacemaker cells |
Muscle membrane depolarizes to threshold level for formation of action potential | Pacemaker cell membranes depolarize to threshold for formation of action potential |
Action potential forms in muscle cell but does not enter other cells | Action potential forms in a pacemaker cell and then propagates from cell to cell throughout the whole heart |
Note: Skeletal muscle cells do not have slow Ca2+ channels | During action potential, extracellular Ca2+ (“trigger” Ca2+) enters cell through “slow” Ca2+ channels |
Action potential causes Ca2+ release from sarcoplasmic reticulum; Ca2+ binds to troponin | Entry of extracellular trigger Ca2+ causes release of more Ca2+ from sarcoplasmic reticulum; Ca2+ binds to troponin |
Actin’s binding sites are made available for actin-myosin cross-bridge formation | Actin’s binding sites are made available for actin-myosin cross-bridge formation |
Cross-bridge cycling generates contractile force between actin and myosin filaments | Cross-bridge cycling generates contractile force between actin and myosin filaments |
Muscle contracts (brief “twitch”); Ca2+ is taken up by sarcoplasmic reticulum | Heart contracts (complete “beat” or “systole”); Ca2+ is taken up by sarcoplasmic reticulum or pumped back out of cell into extracellular fluid |
Muscle relaxes | Heart relaxes |
The Contractile Machinery in Cardiac Muscle Is Similar to That in Skeletal Muscle
Cardiac muscle, like skeletal muscle, has a striated appearance under the light microscope (Figure 19-1). These cross-striations have the same structural basis in cardiac muscle as in skeletal muscle (see Figure 6-2). Each striated cardiac muscle cell (muscle fiber) is made up of a few hundred myofibrils. Each myofibril has a repetitive pattern of light and dark bands. The various bands within a myofibril are given letter designations (A band, I band, Z disk). The alignment of these bands in adjacent myofibrils accounts for the striated appearance of the whole muscle fiber. Each repeating unit of myofibrillar bands is called a sarcomere. This name, which means “little muscle,” is apt because a single sarcomere constitutes the contractile subunit of the cardiac muscle. By definition, a sarcomere extends from one Z disk to the next, a distance of approximately 0.1 mm, or 100 µm.
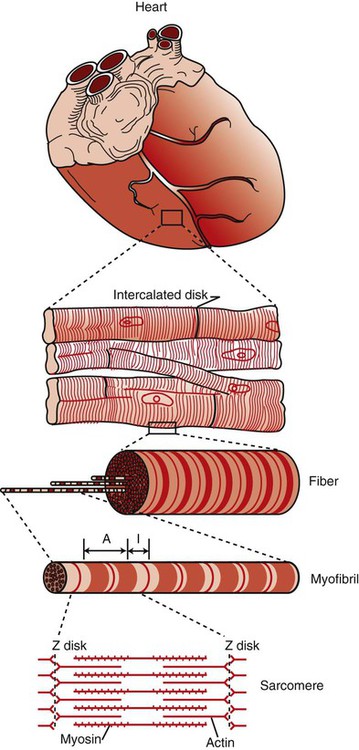
As in skeletal muscle, each cardiac muscle sarcomere is composed of an array of thick and thin filaments. The thin filaments are attached to the Z disks; they interdigitate with the thick filaments. The thin filaments are composed of actin molecules. The thick filaments are composed of myosin molecules. In the presence of adenosine triphosphate (ATP) and calcium ions (Ca2+), myosin and actin interact in a series of steps called the cross-bridge cycle, which results in contraction and force generation in each sarcomere and therefore in the whole muscle cell (for details, see Figures 1-3, 1-4, 1-5, and 6-6).
Cardiac Muscle Forms a Functional Syncytium
Although the molecular basis of contraction is the same for cardiac and skeletal muscle, the two muscle types differ in regard to electrical linkages between neighboring cells, and this difference has important consequences. Individual skeletal muscle cells are electrically isolated (insulated) from one another, so action potentials cannot “jump” from one skeletal muscle cell to another. As described in Chapter 5, an action potential in a skeletal muscle cell is initiated only in response to an action potential in the somatic motor neuron that innervates the skeletal muscle cell. Each neural action potential causes release of the neurotransmitter acetylcholine, which activates nicotinic cholinergic receptors on the skeletal muscle cell, which in turn depolarizes the muscle cell to threshold for the formation of an action potential. When formed, the action potential propagates along the length of that particular muscle cell and then stops. The muscle action potential causes the cell to contract. Neighboring cells may or may not contract at the same time, depending on whether action potentials are initiated in the neighboring cells by their motor neurons.
The specialized cellular structures that allow cardiac action potentials to propagate from cell to cell are evident under the light microscope (see Figure 19-1). Cardiac muscle appears as an array of fibers (individual cardiac muscle cells) that are arranged approximately in parallel but with some branching. Adjacent cells are joined together by dark-appearing structures called intercalated disks. Electron microscopy has revealed that within these disks are tiny open channels between neighboring cells. These nexi, or gap junctions, provide points of contact between the intracellular fluid of adjacent cells. When an action potential depolarizes the cell on one side of an intercalated disk, positive ions flow through the gap junctions and into the neighboring cell. This local, ionic current depolarizes the neighboring cell to threshold for the formation of an action potential. In effect, an action potential propagates from cell to cell through the gap junctions that are located within the intercalated disks. Skeletal muscle does not have intercalated disks or nexi (gap junctions).
Cardiac Contractions Are Initiated by Action Potentials That Arise Spontaneously in Specialized Pacemaker Cells
Because cardiac muscle tissue forms a functional syncytium, and because a cardiac action potential leads to contraction, any one cardiac muscle cell can initiate a heartbeat. In other words, if a single cardiac muscle cell depolarizes to threshold and forms an action potential, that action potential will propagate from cell to cell, throughout the heart, and cause the whole heart to contract. Most cardiac muscle cells have the property of remaining stable at a resting membrane potential; they never form action potentials by themselves. However, a few specialized cardiac muscle cells have the property of depolarizing spontaneously toward the threshold for the formation of action potentials. When any one of these specialized cells reaches threshold and forms an action potential, a heartbeat results. Cardiac cells that depolarize spontaneously toward threshold are called pacemaker cells because they initiate heartbeats and therefore determine the rate, or pace, of the heart.
Although all spontaneously depolarizing cells in the heart are called pacemaker cells, only one pacemaker cell, the one that reaches threshold first, actually triggers a particular heartbeat. In the normal heart, the pacemaker cells that depolarize most quickly to threshold are located in the sinoatrial (SA) node. The SA node is in the right atrial wall, at the point where the venae cavae enter the right atrium.
Because it has spontaneously depolarizing pacemaker cells, the heart initiates its own muscle action potentials and contractions. Motor neurons are not necessary for initiating cardiac contractions, whereas they are needed for initiating skeletal muscle contractions. Motor neurons (sympathetic and parasympathetic) do affect the heart rate, by influencing the rapidity with which the pacemaker cells depolarize to threshold, but the pacemaker cells initiate action potentials, and therefore heartbeats, even without any sympathetic or parasympathetic influences. Thus a denervated heart still beats, whereas a denervated skeletal muscle remains relaxed (in fact, paralyzed). The ability of the heart to beat without neural input enables surgically transplanted hearts to function. When a donor’s heart is connected to a recipient’s circulation during cardiac transplantation, no nerves are attached to the transplanted heart. The pacemaker cells in the transplanted heart initiate its action potentials and contractions. The only factor missing is control of the heart rate through cardiac sympathetic and parasympathetic nerves.
A System of Specialized Cardiac Muscle Cells Initiates and Organizes Each Heartbeat
Each normal heartbeat is initiated by an action potential that arises spontaneously in one of the pacemaker cells in the SA node (Figure 19-2). When formed, the action potential propagates rapidly, from cell to cell, across the right and left atria, causing both atria to contract. Next, the action potential propagates slowly, from cell to cell, through a special pathway of cardiac muscle cells that lies between the atria and the ventricles. This pathway consists of the atrioventricular (AV) node and the first part of the AV bundle, also called the bundle of His. The AV node and AV bundle provide the only route for the propagation of action potentials from the atria to the ventricles. Elsewhere, the atria and ventricles are separated by a layer of connective tissue, which can neither form nor propagate action potentials. In addition to providing the only conductive pathway between the atria and the ventricles, the AV node and the first part of the AV bundle have the special property of very slow conduction of action potentials. It takes 50 to 150 msec for an atrial action potential to travel through the AV node and the first part of the AV bundle; that is, it takes 50 to 150 msec for an atrial action potential to propagate into the ventricles. Slow conduction through the AV junction creates the delay between atrial and ventricular contractions.
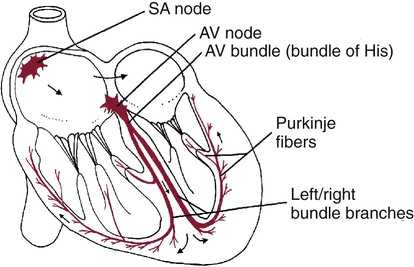
When past the slowly conducting cells of the AV junction, the cardiac action potential enters a branching network of specialized cardiac cells that have the property of extremely rapid propagation of action potentials. The transition zone from slowly conducting to rapidly conducting cells is located within the AV bundle, which has slowly conducting cells in its first portion (connected to the AV node) and rapidly conducting cells beyond that. The rapidly conducting portion of the AV bundle splits to form the left and right bundle branches. At the ventricular apex, the bundle branches break up into a dispersed network of Purkinje fibers, which carry the action potential rapidly along the inner walls of both ventricles. The Purkinje fibers propagate action potentials into the normal ventricular muscle fibers within the inner walls (subendocardial layers) of both ventricles. From there, the action potentials propagate quite rapidly outward, from cell to cell, through the ventricular walls. As the action potential reaches each ventricular muscle fiber, that fiber contracts. The extremely rapid conduction of the cardiac action potential, from cell to cell, through the latter portion of the AV bundle, the bundle branches, and the Purkinje system results in a nearly synchronous contraction of all the fibers in both ventricles.
The SA and AV nodes, AV bundle, bundle branches, and Purkinje fibers are collectively called the specialized conduction system of the heart. This system is composed of specialized cardiac muscle cells, not nerves. The particular characteristics of the components in the specialized conduction system cause each heartbeat to follow a specific, patterned sequence. In a normal beat, both atria contract, almost simultaneously. Next, there is a brief pause (caused by slow propagation of the action potential through the AV node). The two ventricles then contract, almost simultaneously. Finally, the entire heart relaxes and refills.
Figure 19-3 reemphasizes the role of the specialized conduction system in initiating and organizing a normal cardiac contraction. In this “time lapse” illustration, atrial excitation begins at time t = 0, when one SA node cell has reached threshold and an action potential is just beginning to propagate out of the SA node and into regular atrial tissue. Within 0.1 second, the action potential has propagated completely across the right and left atria, and a coordinated contraction of both atria is just beginning. As the action potential propagates across the atria, it also depolarizes the first cells in the AV node, beginning at time t = 0.04 second. While the atria are in a depolarized (excited) state, the action potential is propagating slowly from cell to cell through the AV node and first part of the AV bundle. After traversing this slowly conducting region, the action potential propagates rapidly through the remainder of the bundle of His and its branches. The action potential arrives at the ventricular apex at time t = 0.17 second. Note that it takes about 0.13 second [(0.17 − 0.04) second] for the action potential to travel through the AV node and bundles; that is, 0.13 second represents a typical delay between atrial depolarization and ventricular depolarization. From the ventricular apex, the Purkinje fibers propagate the action potential rapidly throughout both ventricles. Ventricular excitation (depolarization) is complete by time t = 0.22 second, and both ventricles contract. By this time the atria have repolarized to a resting state and are relaxing. After ventricular excitation and contraction, the ventricles relax, and the whole heart remains in a resting state until the next beat is originated by an SA node pacemaker cell.
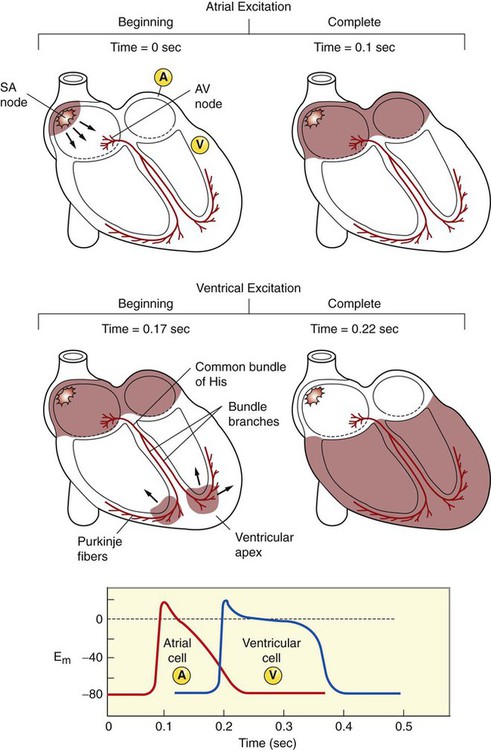
Cardiac Action Potentials Are Extremely Long
Two major differences between action potentials in skeletal muscle and cardiac muscle have already been mentioned: First, action potentials propagate from cell to cell in cardiac muscle, whereas skeletal muscle cells are electrically isolated from one another. Second, the heart has pacemaker cells, which form spontaneous action potentials, whereas a skeletal muscle cell only depolarizes and forms action potentials when “commanded” to do so by its motor neuron.
A third important difference between skeletal and cardiac action potentials is their duration (Figure 19-4). The entire action potential in a skeletal muscle lasts only 1 to 2 msec. A cardiac action potential lasts about 100 times longer (100-250 msec). Prolongation of the cardiac action potential is brought about by prolonged changes in the permeability of the cardiac muscle membrane to sodium, potassium, and calcium ions (Na+, K+, and Ca2+). Cardiac muscle cell membranes have Na+ and K+ channels similar to those found in skeletal muscle, but the timing of their opening and closing is different in cardiac muscle. In addition, cardiac cell membranes also have special Ca2+ channels that are not present in skeletal muscle. The movement of extracellular Ca2+ through cardiac Ca2+ channels has an especially important role in prolonging the cardiac action potential. The presence of Ca2+ channels and the important role of extracellular Ca2+ in the action potential is the fourth major difference between cardiac and skeletal muscle.
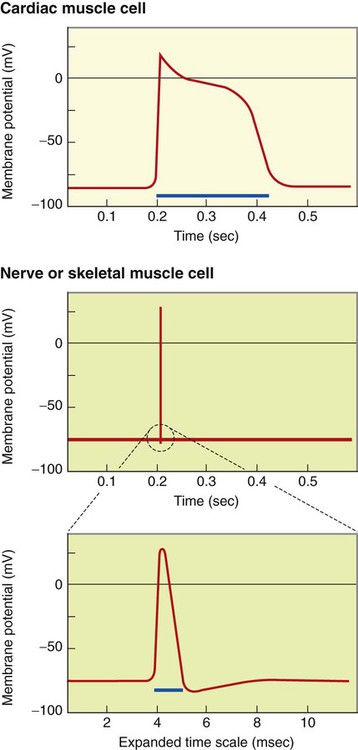
In addition to learning about the special significance of the membrane Ca2+ channels in cardiac muscle, it is useful to review the roles of K+ and Na+ channels in skeletal muscle and to emphasize some ways in which cardiac K+ and Na+ channels are similar to those in skeletal muscle. As explained in Chapter 4, many of the K+ channels in a neuron or skeletal muscle cell membrane are open when the cell is at rest, and most of the Na+ channels are closed. As a result, the resting cell is much more permeable to K+ than to Na+. As a result, there is a greater tendency for positive K+ to exit from the cell than for positive Na+ to enter. This imbalance is the main factor responsible for a resting membrane potential (polarization) in which the inside of the cell membrane is negative in comparison with the outside. The resting membrane potential in skeletal muscle cells is typically between –70 and –80 mV (see Figure 19-4, bottom). An action potential is created when something depolarizes the cell (makes it less negative inside). Specifically, depolarization to the threshold voltage for opening the voltage-gated Na+ channels allows an influx of extracellular Na+ into the cell. This rapid entry of positive ions causes the cell membrane to become positively charged on its inside surface. This positive membrane potential persists for only a moment, however, because the Na+ channels become inactivated very quickly. Na+ entry ceases, and the cell rapidly repolarizes toward its resting membrane potential. Repolarization is also promoted by the opening of additional K+ channels. In fact, this opening of extra K+ channels may cause neurons and skeletal muscle cells to become hyperpolarized (even more negative than normal resting membrane potential) for a few milliseconds at the end of each action potential (see Figure 19-4, bottom).
In a resting skeletal muscle cell, calcium ions are sequestered within the sarcoplasmic reticulum. The occurrence of an action potential in the skeletal muscle cell causes Ca2+ to be released from the sarcoplasmic reticulum into the free intracellular fluid, which is called the cytosol. The increase in cytosolic Ca2+ concentration initiates muscle contraction (see Figure 1-5). The contraction initiated by a single action potential is very brief in skeletal muscle, because the cytosolic Ca2+ is rapidly pumped back into the sarcoplasmic reticulum by active transport, and the muscle relaxes. Note that the Ca2+ responsible for initiating skeletal muscle contraction comes entirely from the intracellular storage site, the sarcoplasmic reticulum. No extracellular Ca2+ enters the cell during the action potential, because skeletal muscle cells do not have membrane Ca2+ channels. In cardiac muscle, in contrast, membrane Ca2+ channels and the entry of extracellular Ca2+ into the cells play key roles in both action potentials and contractions.
Membrane Calcium Channels Play a Special Role in Cardiac Muscle
Figure 19-5 depicts a cardiac muscle cell action potential and the sequence of changes in K+, Na+, and Ca2+ permeability that are responsible for the action potential. As the time line begins (on the left side of each graph), the cardiac cell is at a normal, negative resting membrane potential of about –80 mV. The cardiac membrane potential is negative at rest for the same reason that skeletal muscle cells have negative resting membrane potentials: many K+ channels are open at rest, and most of the Na+ channels are closed. As a result, membrane permeability to K+ is much higher than membrane permeability to Na+ (see Figure 19-5, middle two graphs). In resting cardiac cells, the membrane Ca2+ channels are closed, so Ca2+ permeability is very low (see Figure 19-5, bottom); extracellular Ca2+ ions are prevented from entering the cardiac cells.
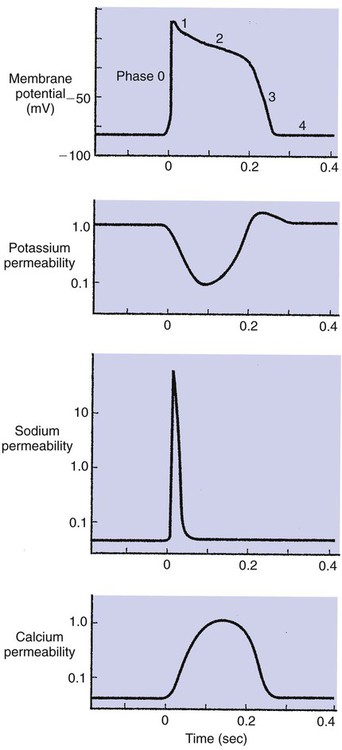
As in skeletal muscle, a cardiac action potential is created when the cell is depolarized to the threshold voltage for opening the voltage-gated Na+ channels. The rapid influx of extracellular Na+ into the cell causes the cell membrane to become positively charged on its inside surface (Phase 0 in Figure 19-5, top). The Na+ channels inactivate very quickly, which causes the Na+ permeability to decrease quickly; the membrane begins to repolarize (Phase 1). However, in cardiac muscle, repolarization is interrupted, and there is a prolonged plateau of depolarization, which lasts about 200 msec (Phase 2). The plateau of the cardiac action potential is brought about by two conditions that do not occur in nerves or skeletal muscle fibers: (1) some K+ channels close, so K+ permeability decreases; and (2) many of the Ca2+ channels open, so Ca2+ permeability increases. Because the Ca2+ concentration is higher in the extracellular fluid than in the intracellular fluid, Ca2+ flows through the open Ca2+ channels and into the cytosol. The combination of reducing the exit of K+ from the cell and allowing the entrance of Ca2+ into the cell keeps the cell membrane in a depolarized state. After about 200 msec, the K+ channels reopen, and the Ca2+ channels close; K+ permeability increases, and Ca2+ permeability decreases. The combination of increasing the exit of K+ from the cell and shutting off the entrance of Ca2+ into the cell causes the cell to repolarize (Phase 3) and eventually to return to its stable, negative resting membrane potential (Phase 4).
The specialized Ca2+ channels in cardiac muscle cell membranes are called slow Ca2+ channels (or L-type Ca2+ channels) because they take much longer to open than the Na+ channels, and they stay open much longer. As shown in Figure 19-5, Na+ permeability increases and then decreases (Na+ channels open and then inactivate) within a few milliseconds. Ca2+ permeability, in comparison, is slow to increase (Ca2+ channels are slow to open) and Ca2+ permeability remains elevated for about 200 msec (the time Ca2+ channels stay open). In recognition of their much quicker responses, the Na+ channels in cardiac muscle are sometimes called fast Na+ channels.
The Ca2+ that enters a cardiac cell during an action potential triggers the release of additional Ca2+ from the sarcoplasmic reticulum. This process is called calcium-triggered calcium release (or calcium-induced calcium release). In less than 0.1 second, the contraction of free Ca2+ in the cytosol increases about 100-fold. As in skeletal muscle, this increase in cytosolic Ca2+ initiates concentration. When the Ca2+ channels close, at the conclusion of the action potential, most of the free, cytosolic Ca2+ is pumped back into the sarcoplasmic reticulum or pumped back across the cell membrane into the extracellular fluid. Both these processes involve active transport, because the Ca2+ is being pumped against its electrochemical gradient. Once the cytosolic Ca2+ concentration is returned to its low, resting level, the cardiac muscle relaxes. Figure 19-6 shows the relationship between action potentials and the resulting contractions in a cardiac muscle cell.
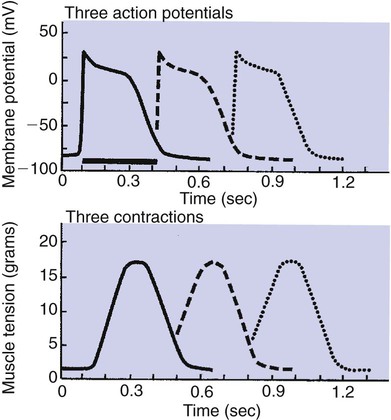
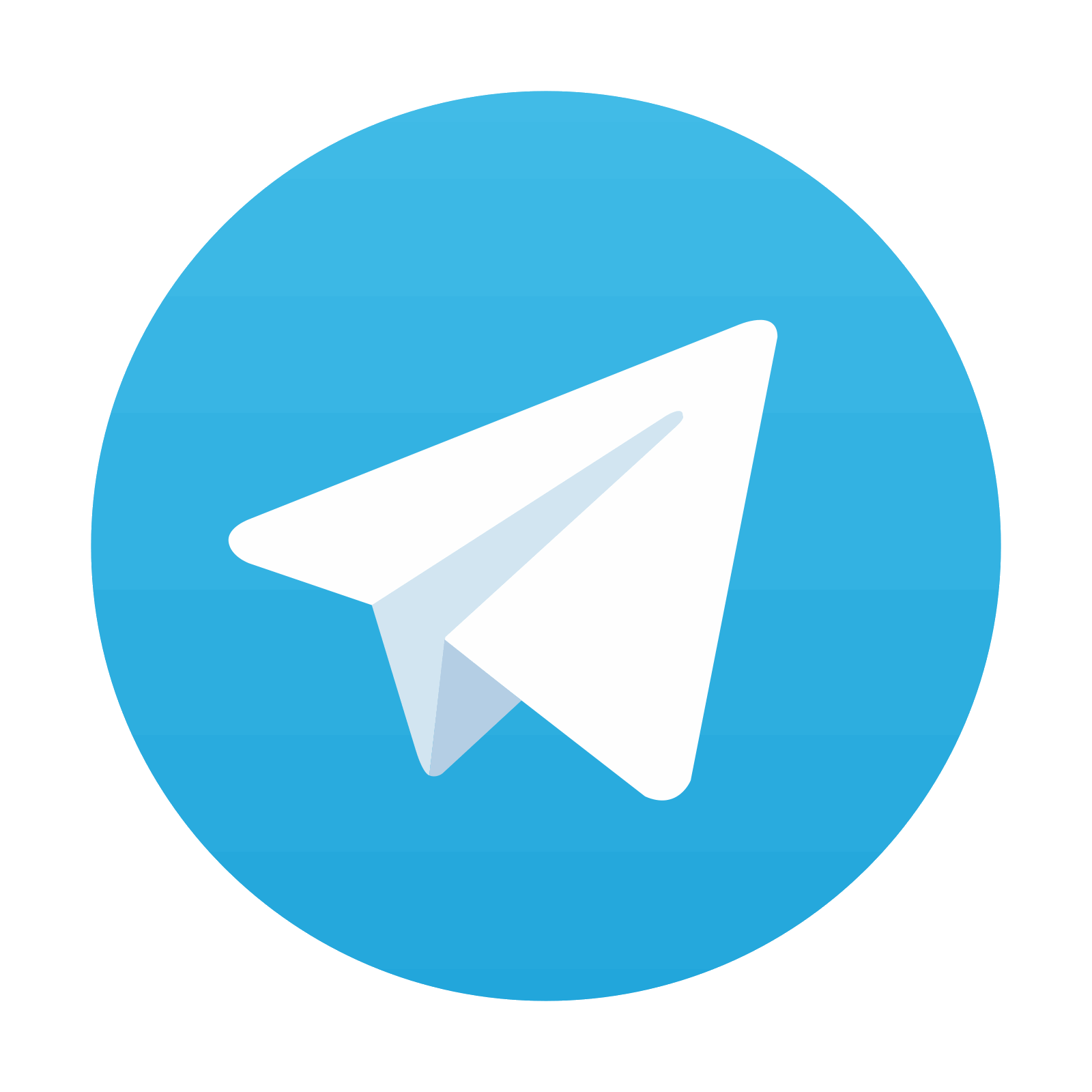
Stay updated, free articles. Join our Telegram channel
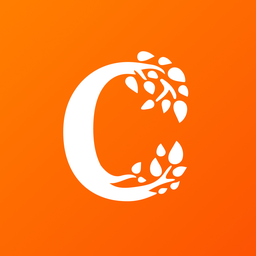
Full access? Get Clinical Tree
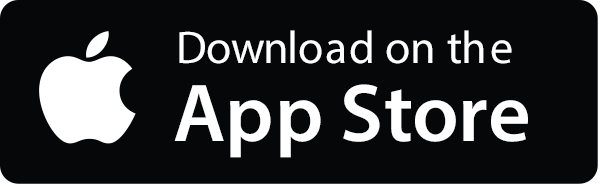
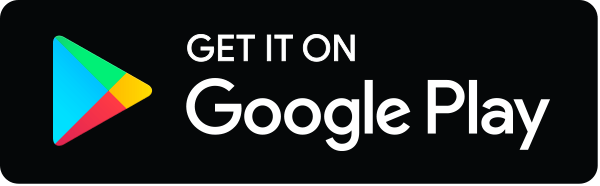