Delayed Unions, Nonunions, and Malunions
General Causes of Delayed Union, Nonunion, and Malunion
Osteosynthesis (healing of bone through surgical intervention with an implantable device) is a complex but robust process whereby the event of fracture initiates a cascade of biologic events directed at reestablishing the mechanical function of the bone. The method of bone healing, primary or secondary (direct or indirect), is influenced by intrinsic factors of the individual patient and fracture, and by extrinsic factors of methods of fracture repair and ancillary treatments. Many strategies and devices have been developed to support or enhance a naturally vigorous healing mechanism. It is useful to divide these strategies into mechanical and biologic. Mechanical strategies aim to realign fracture fragments and attenuate motion at the fracture site to allow healing to occur. Biologic strategies aim to preserve or enhance natural healing processes, which then will ultimately reestablish mechanical function. Combined with these are the four tenets of bone healing and regeneration: mechanics, scaffold, growth factors, and cells.15 If these strategies, whether mechanical or biologic or both, are insufficient, then fracture healing will be retarded (delayed union), unsuccessful (nonunion), or improper (malunion).
Inadequate Mechanical Environment
Mechanics is the study of forces on material systems. In this discourse, mechanics can be divided into the geometric configuration of the healing fracture and healed bone, as well as forces and resultant motion of fracture fragments and the limb. Geometric configuration centers on alignment of the fracture or reduction of fracture segments. The ultimate goal in fracture repair is that a healed and remodeled bone should take on a form and function of the bone as close as possible to that before injury. Included are its length, rotational and angular shape, orientation of adjacent joints, size of the healed bone, and relationship to adjacent soft tissues.
The physical orientation of the fracture fragments is also limited. The fragments must be in appropriately close proximity to each other, so that a healing callus can incorporate and unite two or many sections of the fracture. Too great a gap may overwhelm the healing mechanism. Fracture gap size limits vary, depending on local factors of stability, width of bone, local biologic factors, and preservation or loss of periosteum. In one dog study, nonunion resulted after removal of a 21 mm long segment of diaphyseal bone and periosteum from a femur 14 mm in diameter. In this study, the resulting fracture was rigidly stabilized and gap was maintained with a bone plate.32 Other studies in different bones have created nonunion gap models with varying lengths of fracture gap.20,40,54,55 It is impossible to be definitive with regard to the limits of fracture gaps, but those approaching the diameter of the bone should be avoided.
The second mechanical factor consists of forces and resulting motion of fracture fragments. Naturally occurring bone healing, as evidenced by development of callus in secondary bone formation, is strongly influenced by motion. Motion has effects on developing tissues and can distort, protect, or damage cells. Appropriate motion is an essential part of bone healing, as motion triggers both proliferation and differentiation of stem cells. An extremely stiff fixation minimizes interfragmentary movement, resulting in lesser degrees of callus formation. It is not simply motion, but its direction and magnitude as well, that influences healing. Stem cells respond differently to the direction of applied loads.33 Results of mechanical environments can be observed clinically in the fracture callus of a diaphyseal fracture healing by secondary bony union (Figure 46-1). During weight bearing, most forces across the diaphysis are axial, healing tissues are compressed, and, as with a balloon, tension is created on the outside of the fracture callus. This tension results in low to moderate magnitudes of tensile strain and hydrostatic tensile stress, which is the most favorable mechanical environment for bone formation and is the reason why in callus formation, bone is formed primarily on the outside of the callus in areas of tension and cellular elongation. Closer to the center of the callus, stem cells are subjected more frequently to compressive forces; therefore, chondrogenesis is favored. The same mechanical influences are seen in bone regeneration in distraction osteosynthesis. As the bone segments are distracted, mesenchymal stem cells undergo tensile strain and hydrostatic tensile stress between the fracture ends being distracted. New bone formation is encouraged, and often large amounts of new bone can be regenerated. Exuberant callus outside the central axis is not usually seen.
The magnitude of forces and, more important, the resulting magnitude of motion must be considered. It is essential that strain (deformation) should not exceed the tolerable limit of that tissue, or damage to tissues and cells will result. In the developing fracture callus, fibrous tissue is most tolerant to strain, then cartilage, then bone. As such, if the strain limit of bone is exceeded, osseous tissue would be damaged and cartilage is most likely to form. Subsequently, if the strain limit of cartilage is exceeded, then stem cells are more apt to follow a fibroblastic lineage. Because fibrous tissue conversion to bone is a slow and indirect process, interposition of a preponderance of fibrous tissue in a healing fracture can delay or prevent osteosynthesis.22,23,53
Determining optimal strain has been a quest for orthopedic surgeons and researchers for decades. Strain is a percentage of deformation (see Chapter 41). Axial strain is considered and studied most often in orthopedic surgery, and is the simplest type to understand. Using a common model of study—a gap in a long bone—the amount of strain would be calculated as the decrease in the width of the gap divided by the total width, or a percentage. The amount of strain shown to enhance new bone formation in axial load is as high as 36% in some small gap models, and strains of 7% demonstrate far less bone healing.10 When this is related to clinical situations, it can be seen that small fracture gaps—on the order of 1 mm—can tolerate only small displacements, or axial shortening of 0.3 mm. In contrast, in a comminuted fracture of several or many fragments, the strain would be distributed among many fracture gaps. A highly comminuted area spanning 100 mm could potentially tolerate several millimeters of strain. However, many other factors may influence tolerable strain. The size of the gap has an influence on the effect of strain. Larger strain seems to encourage stronger healing in smaller gaps, and less strain is tolerated in larger gaps. In one study, strains of 30% had a positive effect on the healing of 1 mm diaphyseal gaps compared with 7% strain, but 30% strain had a severely negative influence on bone healing in larger 6 mm fracture gaps, and the lower strain had the better result. For small gaps, larger interfragmentary movements result in larger periosteal areas of callus. For large gaps, interfragmentary movement inhibits stimulation of callus and delays consolidation of the fracture.
Although it is clinically less significant, healing of fractures can be inhibited if too little strain is imparted to the healing fracture callus. This phenomenon is referred to as stress protection. By preventing any stresses from being imparted to the healing callus, the cells of the callus may not have adequate mechanical signaling to propagate and differentiate into functional bone (Figure 46-2). Limiting strain to less than 1% to 2% should be avoided. Ideally, some strain, or motion, should be seen at low loads, then progressively less at higher loads. This would mean low stiffness at loads seen during light motion of convalescence to promote cellular proliferation and differentiation; then as loads increase, the stiffness and strength of fixation would increase, limiting total strain to protect the fracture callus. This nonlinear, increasing stiffness profile is seen in ring fixators and other fixation devises.6,17,31 Again, it is not possible to dictate stringent strain limits as the number of variables is too great. Still, with regard to simple axial loading and fractures with a few to several millimeters of gap or comminution, strains of 5% to 10% are generally considered adequate.42
It is not only the degree of deformation but the orientation of forces on the cells that influences cellular response. Cells of the healing callus undergo compression, tension, or shear. As was noted earlier, mesenchymal stem cells respond to tensile forces by differentiating and proliferating along osteoblastic lineages, and they respond to pure compressive forces by proliferating and after chondroblastic lineages. Compressive and tensile forces are the predominant forces of the skeleton. Bending should be considered a combination of compressive and tensile forces, as structures on the concave aspects of the bend of a beam are under compression, while those on the convex aspect are under tension (Figure 46-3). In pure bending, those in the middle of the beam are neutral. Shear forces also act on fracture gaps and on healing cells. Shear forces are least tolerated, and even modest amounts can be damaging to cells of a fracture callus. This is true for oblique fractures and axial load, and for transverse fractures in rotational loading.16,36 As such, shear movements at the fracture site worsen the healing process compared with compressive movements, resulting in decreased periosteal callus, delayed bone formation, and inferior mechanical stability (Figure 46-4).1
Figure 46-4 Lateral radiograph of a femur 12 weeks postfracture repair using an interlocking nail. This type of nail allowed rotational instability at the fracture site, resulting in high shear force. The fracture components repaired with cerclage wire have healed, whereas the area of fracture subjected to rotational and shear forces has progressed to nonunion.
Inadequate Biologic Environment
Biologic factors of bone healing can be simply divided into growth factors and cells, although certainly these are intimately related. The litany of growth factors active in bone healing is large and continues to expand. The role of growth factors, most notably the bone morphogenetic proteins (BMPs), has been extensively studied and reviewed, with BMP-2 known to be essential.30,56 Growth factors are initially released from the intercellular matrix. Hemorrhage and platelet degranulation further deliver growth factors and cytokines to the fracture area, initiating a cascade of humoral influences that are linked to cellular activity not only of bone, but of fibrous tissue and vasculature. Cellular activity, including migration, adhesion, proliferation, and differentiation, occurs in concert with humoral signaling.19 It is important to note that growth factors are active in a very specific temporal orchestration with the concentration of each waxing and waning in order. Some are active for only brief periods, and subsequent response of cellular activity or further expression of growth factors is contingent on preceding expression and activity. Considerable redundancy has been noted in growth factor influence on bone healing, but still, disruption of one set of signaling mechanisms early in bone healing may have extended consequences and may play a role in delayed unions and nonunions. Because growth factors are released initially from the local fracture environment, then are perpetuated by continued cellular activity, enhancing growth factor influences should concentrate on preserving the local fracture environment and promoting a positive cellular environment.
Views on preserving the local fracture area have long been appreciated and are receiving more attention with improved understanding of fracture biology. Minimally invasive techniques that preserve soft tissue viability and local vascularity are commonly reported and employed.24,37,43,45–47,50,52 These techniques preserve the local fracture environment, which thereby preserves the presence and activity of growth factors and promotes a cellular response. In contrast, techniques that remove or otherwise compromise humoral influences can delay fracture healing.
Intrinsic and extrinsic negative influences of the patient and the fracture on the local growth factor environment need to be appreciated. Diaphyseal cortical bone, decreased/compromised vascularity of the periosteum, sparse soft tissue attachments, and an aged patient all are negative intrinsic factors for growth factor activity.* Extrinsic factors include those imposed by surgical decisions or techniques. Open reduction and internal fixation invade the fracture environment. The surgical approach elevates soft tissues, and lavage removes the fracture hematoma with its cellular and humoral components of the early soft tissue response. Some devices are less biologic than others, and bone plates luted† to bone may have a negative effect.12,41 Reaming of bone compromises medullary blood flow.9,26 The temporal nature of growth factors and of bone healing should be appreciated. Initial healing activity and cytokine signaling seen early in fracture healing will not be maintained after weeks to months. If activity is not seen or is thwarted in the first few weeks of bone healing, it cannot be expected to be expressed later without strategies to reintroduce an early fracture healing environment.
Cellular activity is inextricably related to growth factor expression and activity, and the requirements for an adequate or insufficient environment are the same. The principal cell type is the mesenchymal stem cell.3–5,33 These cells follow osteoblastic or chondroblastic lineages, depending on the local environment. The cambium layer of the periosteum is an important source of stem cells and intuitively places them in a fracture location as a ready source for recruitment. This population is richest in areas of thick and vascular periosteum. Mesenchymal stem cell activity is greatest in youth and tends to degrade in older age.4 A rare but important population of mesenchymal stem cells is seen in bone marrow, often in the metaphyseal bone areas. These cells proliferate most and differentiate down osteoblastic lineages best when exposed to proper growth factors, vascular and oxygen-rich environments, and proper mechanical deformation. Fractures that occur in environments of thick periosteum, metaphyseal bone, young patients, high vascularity, and modest hydrostatic tension have the greatest cellular activity.49
In contrast, cellular activity is least in diaphyseal bone with limited soft tissue attachments, limited medullary space, and motion that exceeds the mechanical limit of the early fracture callus. A common example of a hostile environment for stem cell activity is transverse or short oblique radial and ulnar fractures in small-breed dogs (Figure 46-5). Here vascular soft tissue coverage or attachment of muscles in this area is scant. Periosteum has low cellular activity. The medullary space and corresponding medullary vascular resources are limited, sometimes severely.58 The requirements for an adequate mechanical environment, limiting the strain to very tight tolerances, are very high because the bone and the fracture gap are very small. Cellular activity seen in these fractures is often meager and delayed, and nonunion is common.
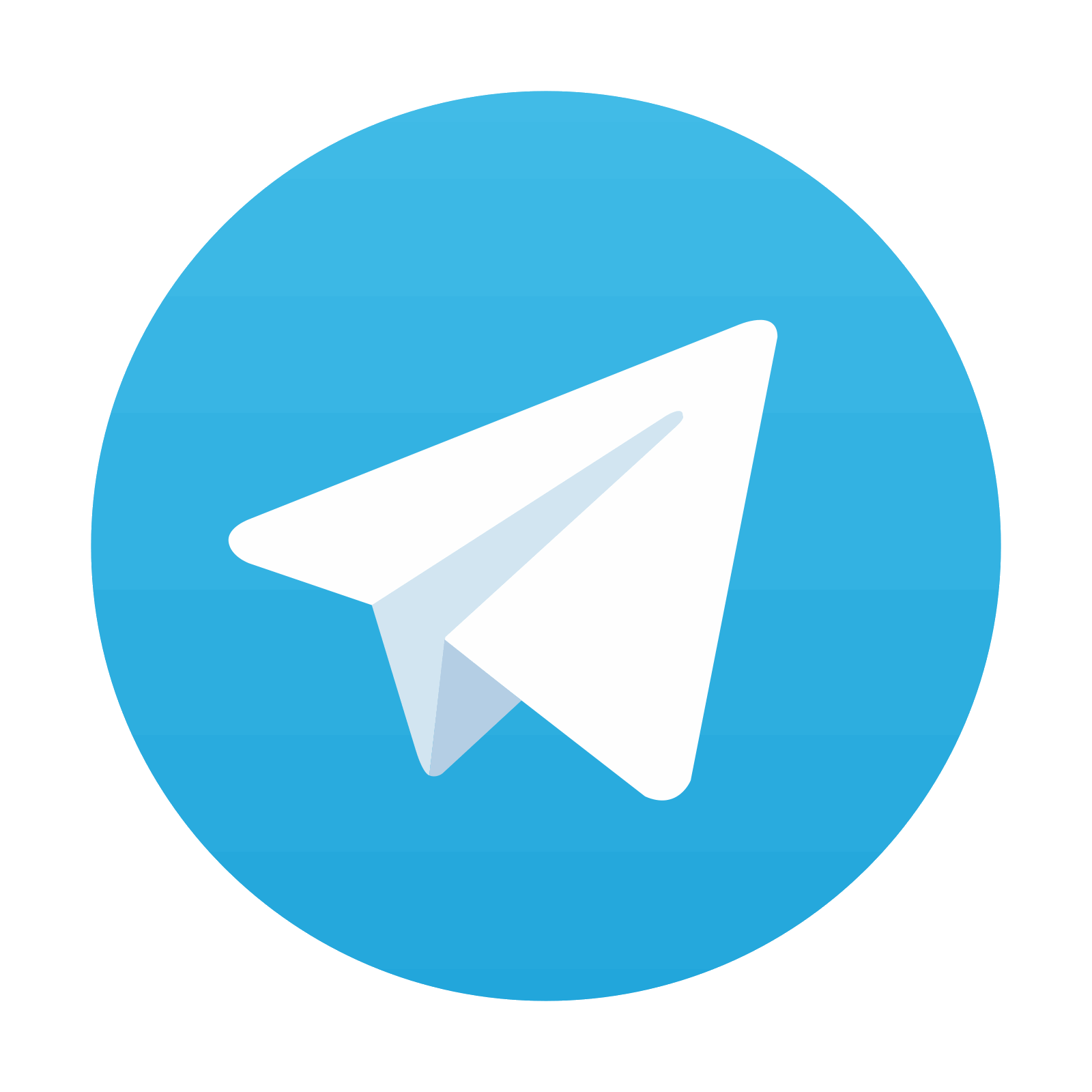
Stay updated, free articles. Join our Telegram channel
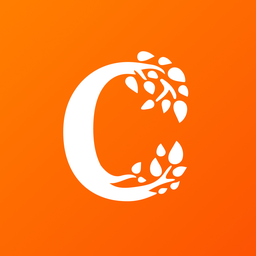
Full access? Get Clinical Tree
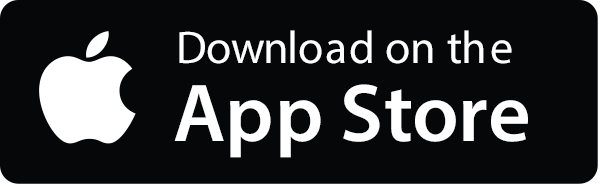
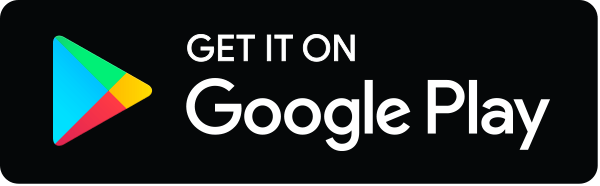