Fig. 3.1.
International Classification of Functioning, Disability, and Health (modified from World Health Organization 2001).
Table 3.1
Classification of test and measures
Test and measure | Clinical | Laboratory | Body structure and function | Activity |
---|---|---|---|---|
Retropulsion test | x | Postural instability | ||
Turning | x | Freezing Bradykinesia | Turning while walking | |
Timed UpandGo | x | Functional mobility (sit-to-stand, ambulation, turning while walking) | ||
Berg Balance Scale | x | Postural control Sensory integration | Functional mobility (sitting, standing, sit-to-stand, reaching, stepping, turning) | |
Unified Parkinson’s Disease Rating Scale | x | Tremor Rigidity Bradykinesia Rapid alternating movement Speech Posture Postural control | Ambulation Sit-to-stand Activities of daily living | |
Electromyography | x | Tremor | ||
Quantitative digitography | x | Bradykinesia Freezing | ||
Motion analysis | x | Bradykinesia Tremor Gait and reach spatiotemporal characteristics Postural control | ||
Force platform perturbation | x | Postural control |
2 Clinical Assessment Tools
2.1 Body Structure and Function Level Measures
2.1.1 Retropulsion Test
The retropulsion test is a quick, simple test used by clinicians to assess postural instability. The test involves a posterior pull on the patient’s shoulder and observation of the balance reaction, although there are many variations which differ in rating methods and the expectedness of the perturbation (1). A cross-sectional study that examined different techniques such as expected versus unexpected perturbation concluded that an unexpected pull rated on the 4-point ordinal scale proposed by Nutt and colleagues appears to have greatest predictive values for risk of falls (1). However, the test must be interpreted with caution as it has only been shown to have moderate correlation with performance on platform perturbation (see Section 3 under laboratory tests and measures) during OFF state and no significant correlation during ON state (2) Table 3.2.
Rating | Description |
---|---|
0 | Normal, may take two steps to recover |
1 | Takes two or more steps; recovers unaided |
2 | Would fall if not caught |
3 | Spontaneous tendency to fall or unable to stand unaided (test not executable) |
2.1.2 Turning
Turning is a common, but complex postural control task because it requires that an individual initiate disequilibrium necessary to produce the turn during ongoing movement (3). While persons within 1 year of diagnosis of Parkinson’s disease typically show minimal deficits of gait performance in a single direction, they often report difficulty with turning tasks (4, 5). Several studies that have examined turning in individuals with PD have utilized (1) counting the number of steps required for completing a 180° turn either on the spot or around an obstacle (making a u-turn); (2) quantifying the time it takes to complete a turn; (3) observing step length; and (4) observing the turning arc. Greater number of steps, increased time to complete a turn, as well as decreased step length and wider turning arc in individuals with PD have been observed (6, 7).
2.1.3 Unified Parkinson’s Disease Rating Scale (UPDRS)
The UPDRS is the gold standard assessment most commonly used by both neurologists and researchers to determine disease severity and progression. It was developed in 1987 by a group of movement disorder specialists who derived components of existing PD rating scales to create a single tool that would allow for easier comparison of assessment (8). The test is partly based on patient/caregiver interview and partly on clinical testing by an examiner. It consists of six main sections: (1) mentation, behavior, and mood, (2) activities of daily living (ADLs), (3) motor, (4) motor complications, (5) modified Hoehn & Yahr staging, and (6) Schwab and England activities of daily living scale. A total score of 199 is possible on the first four subscales, with 0 representing no involvement and 199 representing severe disease. Within the context of the organization of this chapter, the UPDRS consists of elements that address both the body structure and function and activity levels of the ICF framework. Specifically, Section 2 (ADLs) predominantly addresses the activity level while Section 3 (motor) focuses on the body structure and function level, with a few items examining the level of assistance required to perform a task, therefore extending to the activity level (Table 3.3).
Table 3.3
Sample items from the Unified Parkinson’s Disease Rating Scale motor subscale [Fahn et al. (115)]
Item | Scoring |
---|---|
Tremor at rest | 0 = absent 1 = slight and infrequently present 2 = mild in amplitude and present most of the time 3 = moderate in amplitude and present most of the time 4 = marked in amplitude and present most of the time |
Rigidity | 0 = absent 1 = slight or detectable only when activated by mirror or other movements 2 = mild to moderate 3 = marked, but full range of motion easily achieved 4 = severe, range of motion achieved with difficulty |
Gait | 0 = normal 1 = walks slowly, may shuffle with short steps, but no festination (hastening steps) or propulsion 2 = walks with difficulty, but requires little or no assistance; may have some festination, short steps, or propulsion 3 = severe disturbance of gait, requiring assistance 4 = cannot walk at all, even with assistance |
Postural stability | 0 = normal 1 = retropulsion, but recovers unaided 2 = absence of postural response; would fall if not caught by examiner 3 = very unstable, tends to lose balance spontaneously 4 = unable to stand without assistance |
Body bradykinesia and hypokinesia | 0 = none 1 = minimal slowness, giving movement a deliberate character; could be normal for some persons. Possibly reduced amplitude 2 = mild degree of slowness and poverty of movement which is definitely abnormal. Alternatively, some reduced amplitude 3 = moderate slowness, poverty, or small amplitude of movement 4 = marked slowness, poverty, or small amplitude of movement |
The UPDRS motor subscale includes 19 items examining speech, facial expression, resting and active tremor, rigidity, rapid alternating movement, arising from a chair, posture, gait, postural stability, and bradykinesia. Each question is rated on an ordinal scale of 0 (normal)–4 (severe limitation) based on qualitative descriptions. Action and postural tremor of hands, arising from a chair, gait, and postural stability are four items within the motor scale that span the body structure and function and activity levels. They examine characteristics of the movement, such as speed and amplitude (body structure and function level), as well as interference with function and assistance required for safe performance (activity level). Studies that have examined the psychometric properties of the motor subscale suggest high internal consistency (Chronbach’s alpha 0.88–0.91) (9, 10), interrater reliability (ICC = 0.82) (11), and test–retest reliability (ICC = 0.90) (12). The motor subscale has moderate-to-good correlation with Hoehn & Yahr classification (H&Y), a staging instrument based on progression of PD, and with timed functional performances (9, 10, 13).
Recently, the Movement Disorders Society (MDS) reviewed the clinimetric properties of the UPDRS and published a revised MDS-UPDRS. Several changes have been made to the motor subscale, including specific items added to address toe-tapping, freezing in gait, constancy of resting tremor, as well as more detailed instructions and descriptions in effort to better standardize test administration and grading (14). The internal consistency remains high (Chronbach’s alpha = 0.93) and further studies need to be performed to determine the reliability of the MDS-UPDRS (14).
2.2 Activity Level Measures
2.2.1 Timed Up- and-Go
The Timed Up-and-Go (TUG) test was developed by Podsiadlo and Richardson to assess basic mobility skills in frail community-dwelling elderly (15). The patient is instructed to stand up from an armchair, walk a distance of 3 m, turn, walk back, and sit down. A stopwatch is used to determine the amount of time required to perform this task and interpretation is made based on the average of three trials (15). Although there exists some controversy in the literature (16) the vast majority of studies suggest excellent (ICC = 0.81–0.99) test–retest reliability and interrater reliability for the TUG in elderly adults (15, 17, 18). TUG times demonstrate correlation with the Berg Balance Scale (r = −0.81), gait speed (r= −0.61), and Barthel Index of activities of daily living (r = −0.78) (15). This functional mobility test has been shown to be a sensitive and specific tool to predict fall risk in elderly adults, with a score higher than 13.5 s indicating high risk of falls (19).
Several studies have examined the use of TUG in populations other than elderly adults, including individuals with strokes, PD, arthritis, and amputations. The psychometric properties of the TUG when applied to patients with PD appear to closely mimic those observed in elderly adults. Interrater reliability has been shown to be good (ICC ≥ 0.73) regardless of whether the patient is ON or OFF medication and internal consistency is high (r = 0.90–0.97) (20). The TUG scores demonstrate significant difference during ON and OFF states, suggesting usefulness in detecting change in medication-related change in status in individuals with PD (20). The minimal detectable change (MDC) using a 95% confidence interval (MDC95) has been reported to be 1.63 s (21).
In recent years, there has been a growing interest in learning about dual task performance in individuals with PD. A variation of the TUG that includes a high level, concurrent cognitive task (TUGcog) (counting the days of the week backward) has been used to assess the interaction of cognitive demand on functional mobility. Individuals with PD demonstrate significant increase in their TUGcog time and number of steps taken during the test – whereby healthy older adults do not demonstrate such changes (22).
2.2.2 Berg Balance Scale
The Berg Balance Scale (BBS) is a 14-item test that was initially developed to assess balance in the geriatric population. Activities examined include standing (with eyes open, eyes closed, feet close together, feet in tandem, single leg), sitting, transfers (sit-to-stand, chair-to-chair), turning (360° in place, weight shift without moving feet), alternate stepping, and reaching (level and to the floor). The test requires two standard height seating surfaces (one with arm rests, both approximately 18″ high), a yard stick, a stopwatch, and an average height foot stool or step (approximately 7″). Specific instructions are provided to increase standardization and administration time ranges from 15 to 20 min, depending on examiner’s proficiency and the patient’s level of function. Each item is rated on a 0–4 ordinal scale, with 0 representing poor ability to perform task. The BBS has been shown to be a valid and reliable test for predicting falls in community-dwelling adults, as well as length of stay, motor function, and disability level in individuals post-stroke (23–26).
Current research supports the use of the BBS as an ongoing assessment tool to determine fall risk and functional mobility in individuals with PD. It has good internal consistency (ICC = 0.86–0.88) and demonstrates moderate-to-high correlation (Spearman correlation = 0.50–0.78) with other functional measures (the forward and backward functional reach test, and Timed Up-and-Go, normal and fast gait speed), as well as with the UPDRS motor subscale (27–29). The MDC95 has been reported to be 5 points (29). Different cut-off scores have been proposed to maximally increase specificity and sensitivity of the BBS in this population to detect falls (43.5 and 54, respectively) (24, 30). Regardless of which cut-off score is used, it is important to consider the multifactorial nature of falls and examine personal (physical, cognitive, emotional, medication use, co-morbidities) and contextual factors (home environment, social support available) to most accurately predict fall risk.
3 Laboratory Assessment Tools
3.1 Body Structure and Function Level Measures
3.1.1 Electromyography (EMG)
EMG has been used to characterize upper extremity tremor presentation, specifically tremor occurrence, symmetry, frequency, and intensity, in individuals with PD. Long-term recording (up to 24 h) has been used for both differentiation of parkinsonian tremor from essential tremor, as well as for assessment of medication efficacy (31, 32). Forearm tremor can be recorded using surface electrodes and a commercial portable recorder, with electrode placement on the wrist or finger extensors (extensor carpi radialis, extensor digitorum) and wrist or finger flexors (flexor carpi ulnaris, flexor digitorum superficialis) (27, 33). The advantages of using solely EMG to quantify tremor include portability and the ability to obtain tremor recording without need to restrict limb movement, as would be necessary if using accelerometry (32). However, often times researchers seek to obtain both neuromuscular and kinematic information, therefore EMG is utilized in conjunction with motion analysis (31, 33, 34).
3.1.2 Quantitative Digitography (QD)
Researchers have used quantitative digitography as a technique to quantify bradykinesia in individuals with PD. The system, adopted from the electronic music industry, consists of a portable keyboard with optical sensors and a computer interface (called musical instrument digital interface, MIDI) (35, 36). The sensors are able to identify the key struck, time of strike and release, and velocity of strike, thereby allowing determination of the frequency and duration of digital movement (35).
Bronte-Stewart and colleagues utilized QD to examine digital control in individuals with idiopathic PD. They determined the technique to be useful in providing objective measures for bradykinesia, fatigue, and freezing during a 60-s alternating finger-tapping task (35). Performance was significantly different in subjects ON and OFF medication (35). QD has also been used to assess improvements in bradykinesia after microelectrode recording in patients undergoing deep brain stimulation surgery (37).
3.1.3 Motion Analysis (MA)
Motion analysis is commonly used to quantify movement kinematics and has been applied to the examination of bradykinesia, tremor, reaching, gait kinematics, and postural control in PD research (33, 38–41). Common methods employed include accelerometry, motion analysis system, and the GAITRite system. Accelerometry is a method used for motion analysis which involves application of acceleration sensors on a specific body part of interest, such as on the L3 spinous process for measuring trunk acceleration or on the forearm for measuring upper extremity bradykinesia. Depending on the type of sensor, signals produced are proportional to the linear acceleration or angular velocity of movement (37, 42). Selection of a sensor is based on relative frequency of the movement measured as well as the range of dynamic movement (42). The use of accelerometry for quantifying spatiotemporal gait kinematics, including gait speed, step length, and cadence in individuals with PD, has been shown to have good concurrent validity with measurements obtained using the GAITRite system (43). Reliability and validity of accelerometry for measuring gait speed, cadence, stride length, single/double limb support times, swing time, and stance time have been established across various gait speeds in healthy adults and individuals with hemiparesis (44). The root mean square velocity of angular movement is indicative of average speed and demonstrates significant inverse correlation with the bradykinesia subscore on the UPDRS (37). This technique has been used to study bradykinesia in forearm supination and pronation (41).
Motion analysis systems, such as Vicon®, involve placement of infrared-emitting markers on landmarks of interest and capturing movement with use of a motion capturing system, often consisting of multiple cameras and specific processing software. This particular technique allows for calculation of spatiotemporal parameters (magnitude, duration, onset, frequency of movement), movement trajectory, estimation of joint centers, and center of mass (COM) changes during movement. There is evidence that the accuracy of this technique may vary, depending on camera set-up, marker size, and lens filter application (45). Motion capturing systems have been used to examine reach velocity and accuracy during ON/OFF medication status (38), tremor after subthalamic deep brain stimulation surgery (34), head–trunk rotation during turning (46), and the relationship between alterations in gait kinematics and disease severity (13).
The GAITRite system is specific for measurement of gait performance. It consists of a 4.6-m electronic, portable walkway in which an array of 16,128 sensors is embedded and organized in a grid-like pattern to identify foot contact during gait (47, 48). It is connected to a computer and generates spatiotemporal recordings, including cadence, step time, step length, mean normalized velocity, step length ratio, heel-to-heel base of support, and single and double limb support time as a percentage of the gait cycle (48). The GAITRite system demonstrates the ability to differentiate gait performance in individuals with PD compared to healthy controls, as well as detect changes in gait parameters during ON and OFF medication states that correlate with changes in UPDRS motor section scores (47). It has been recommended as a tool to assist monitoring and reassessment of treatment efficacy (48).
In conjunction with a motion analysis system, use of force platforms embedded in the floor can provide ground reaction force and center of pressure (COP) data to measure postural control (i.e., COP displacement) during gait initiation, gait termination, and turning (49, 50). An emerging method for assessing dynamic postural control in PD is to measure the anterior–posterior and medial–lateral distance between the extrapolated center of mass (eCOM) and center of pressure (COP) [see (51) for specific method]. Simply stated, the greater the distance between eCOM and COP the more the movement is facilitated. However, these conditions require more active postural control to counteract the disequilibrium. Therefore an individual can be stable through smaller differences between eCOM and COP, but at the expense of movement (i.e., too stable); conversely an individual can produce movement with greater distance between eCOM and COP but at the risk of falling. The ideal situation then is to have a “controlled disequilibrium” in order to facilitate movement with control. Our data from a recent pilot study suggest that while measures of gait performance using motion analysis were not sensitive enough to differentiate between individuals with early PD (within 3 years of diagnosis) and age-matched healthy control subjects, significantly decreased distance between eCOM and COP during turning tasks was observed in our subjects with PD (Song et al., unpublished findings, 2008 (52)).
3.1.4 Force Platform Perturbation (FPP)
Individuals with PD frequently report difficulty with balance and frequent falls. An objective measure used to quantify dynamic postural control involves use of a platform that can provide perturbation via multidirectional linear translations and tilts. Subjects stand on a force plate that is embedded in the platform to provide information on center of pressure changes. FPP has been used alone and in conjunction with EMG to quantify a number of different aspects of postural control, including (1) muscle activation pattern; (2) magnitude of muscle response; (3) latency of muscle response; (4) direction of ground reaction force; and (5) magnitude of ground reaction force (2, 53–55). Although these studies utilize sophisticated equipment set-ups, a commercially available product – the SMsART Balance Master® – has become increasingly popular in the clinical setting and has enabled examination and monitoring of postural control changes in patients with PD.
4 Morphological and Neurochemical Correlatesto Changes in Locomotion in the Rat 6-Hydroxydopamine Model of Parkinson’s Disease
Although it is well established that in Parkinson’s disease there is a significant loss of dopamine within nerve terminals in the striatum, the depletion of dopamine most likely influences other neurotransmitter systems. There is growing interest in the interactions between dopamine and glutamate and it may be the lack of dopamine in Parkinson’s disease that results in dynamic changes in glutamate within at least the striatum (56, 57). In the rodent, the sensorimotor cortex provides the primary excitatory, glutamatergic input to the dorsolateral striatum (58, 59). This projection utilizes the vesicular glutamate transporter-1 (VGLUT-1). Recent data suggest that glutamate input from many nuclei within the thalamus may also be playing an important role (60–62). This thalamostriatal projection utilizes the vesicular glutamate transporter-2 (VGLUT-2). The dopamine terminals originating from the substantia nigra pars compacta make a symmetrical synaptic contact not only on the dendritic shaft of the medium spiny neuron but also on the neck of the dendritic spine (63, 64). The asymmetrical synaptic contact on the head of that same spine within the dorsolateral striatum originates from not only the motor cortex but also the thalamus (60, 62) and the nerve terminals contain the neurotransmitter, glutamate (63, 65, 66). Not only are dopamine and glutamate terminals anatomically located next to each other, these two neurotransmitters can control their own release and also the release from each other’s nerve terminals (67–70). In addition, a small percentage of the glutamate nerve terminals originating from the cortex contain presynaptic dopamine D-2 receptors (71, 72). When these dopamine D2 receptors are activated or blocked, we and others have reported that glutamate release decreases or increases, respectively (67, 69, 73). Therefore, alterations in the level of striatal dopamine can have profound effects on nearby glutamate synapses.
In rodent models of nigrostriatal dopamine loss, either using the neurotoxins 6-OHDA or MPTP, exercise is neuroprotective and enhances behavioral recovery from injury (74). Immediately following the unilateral loss of striatal dopamine, rats were forced to use the impaired limb by the placement of the unimpaired limb in a cast (75). Improvement in the use of the impaired limb occurred, leading to increased levels of striatal dopamine compared to the non-casted animals. However, it was critical that the casting occur within a short-time frame after the nigrostriatal lesion (75). This same group reported, using the MPTP mouse model, that treadmill exercise started within 24 h of the MPTP lesion attenuated the loss of striatal dopamine and the motor behavioral deficits compared to the lesioned but non-exercised group (74). Following intracerebral injection of 6-OHDA into the striatum, if treadmill exercise was initiated up to 7 days post-lesion, there was some recovery of striatal dopamine levels and a decrease in the number of apomorphine-induced contralateral rotations (76). However, 7 days is a time point in which the loss of dopamine neurons within the substantia nigra is not complete (77). Other measures of behavioral deficit were not improved by exercise. It must be recognized that in all of the above studies, exercise was initiated prior to the complete loss of striatal dopamine as a model of neuroprotection. In a recent study in which exercise was initiated just after intrastriatal injection of 6-OHDA, there was significant behavioral/motor improvement but no change in the number of dopamine cells remaining in the substantia nigra or dopamine transporter levels in the striatum (78). This suggests that other neurotransmitters, such as glutamate, could be compensating for the loss of striatal dopamine in terms of locomotor improvement.
The effect of physical activity in both rats and mice on changes in striatal glutamate synapses following the loss of nigrostriatal dopamine is of particular interest in terms of how whether such therapy may be able to further compensate for the loss of dopamine cells in the substantia nigra. In a mouse model of partial bilateral nigrostriatal dopamine loss, treadmill exercise was initiated 4 days after the acute administration of the neurotoxin, MPTP, a time point at which dopamine cell body death has been completed (79). We find that exercise improved motor performance of the MPTP-treated group and resulted in a reversal in the changes in striatal nerve terminal glutamate immunolabeling to the level observed in the control group. In this same study, exercise resulted in a further decrease in striatal dopamine transporter immunolabeling compared to the lesioned but non-exercised group (79).
We used a combination of in vivo microdialysis and quantitative immunogold electron microscopy to determine changes in striatal glutamate following a lesion of the nigrostriatal pathway, followed by exercising the rats for 4 weeks. However, there is controversy as to the origin of the basal levels of glutamate that are measured in brain and whether this extracellular glutamate is derived from the (1) calcium-dependent vesicular pool, (2) calcium-independent, cytoplasmic pool associated with the glutamate/cystine antiporter, or (3) glial pool (80). We and others have reported that about 30% of basal extracellular glutamate is calcium dependent (80–82) and that over 60% of the K+-depolarized extracellular level of glutamate is calcium dependent (83). This suggests a role for the synaptic vesicle pool within the nerve terminal contributing to the extracellular level of glutamate. Replacement of calcium with the divalent chelating agent, EGTA, and increasing the concentration of magnesium resulted in a decrease in the baseline level of glutamate (81), suggesting that a portion of the resting level of striatal glutamate is of neuronal and not glial origin. Therefore, not only is the basal level of glutamate measured, but also using ultrastructural immunocytochemistry, we quantify the relative density of glutamate immunogold labeling within nerve terminals making an identified asymmetrical (excitatory) synaptic contact. In addition, we have developed a double-labeling procedure in which the nerve terminal is first labeled with an antibody against either the vesicular glutamate transporter 1 or 2, followed by immunogold labeling for glutamate.
In this particular rodent model of Parkinson’s disease, rats are administered the neurotoxin 6-hydroxydopmaine (6-OHDA), into the medial forebrain bundle on one side of the brain, in order to destroy the dopamine pathway from the substantia nigra to the striatum (83). This results in a greater than 90% loss of dopamine levels in the striatum and a nearly complete elimination of dopamine cells in the substantia nigra pars compacta (81, 83, 84). In order to determine the effects of exercise on motor behavior related to the loss of dopamine neurons, two tests were performed. The loss of dopamine on one side of the striatum will result in an increase in dopamine receptors on that side of the striatum (denervation supersensitivity). Administration of dopamine-receptor agonist drugs, such as apomorphine, will result in the animals turning away from the side of the lesion (contralateral rotations) (Fig. 3.2). This is a measure of the loss of dopamine/dopamine function and of turning behavior. We find that in the 6-OHDA-lesioned rats, exercise results in a significant decrease in the number of contralateral rotations compared to the lesioned but non-exercised group (Fig. 3.2). As another measure of motor function, exercise results in an increase in the time the rats can run on the treadmill at a maximum speed of 18 m/min (Fig. 3.3).
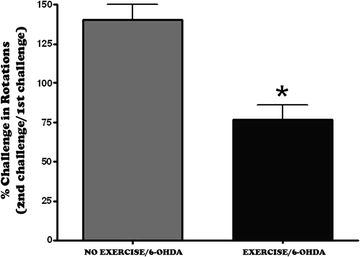
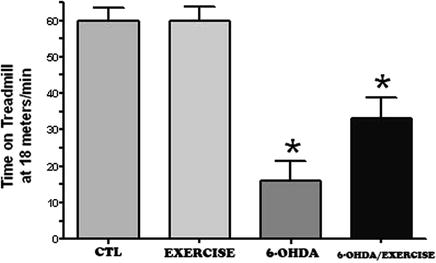
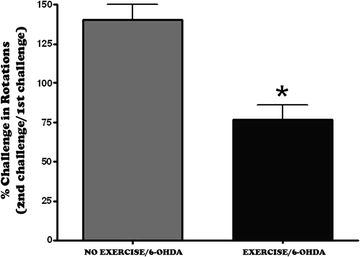
Fig. 3.2.
Exercise decreases apomorphine-induced contralateral rotations. Two months following a 6-OHDA lesion, the animals were injected with apomorphine (0.05 mg/kg, s.c.) and the number of contralateral rotations counted (first challenge with apomorphine). One month following exercising of the rats (1 h/day, 5 days/week), all the animals were tested for apomorphine-induced contralateral rotations (second challenge with apomorphine). The percent change in contralateral rotations between the second and first challenge (i.e., number of rotations at second challenge/number of rotations at first challenge) was then determined. An overall group mean was calculated (mean percentage ± SEM) and the lesioned but no exercise group compared against the lesioned plus exercise group using the student’s t-test. * p <0.05 compared to the other group.
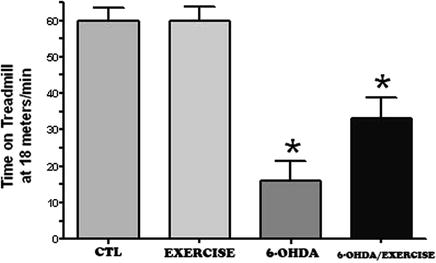
Fig. 3.3.
Exercise increases the time running on the treadmill. Two months following a 6-OHDA lesion or sham lesion (control), the animals exercised for 1 h/day for 1 month (5 days/week). At the end of the 1-month time period, the rats were tested on the treadmill at the maximum speed of 18 m/min to determine how long the rats could stay on the treadmill. The time it took for them not to be able to maintain their running was determined. * p <0.05 compared to all other groups using an ANOVA, followed by Tukey–Kramer for comparison of multiple means.
The data suggest that following the loss of dopamine, there is a significant decrease in the extracellular levels of striatal glutamate (Fig. 3.4). We also demonstrate that, using quantitative immunogold electron microscopy (Fig. 3.5) there is an accumulation of glutamate within the nerve terminals of the striatum (Fig. 3.6), inversely correlating with the decrease in extracellular glutamate as seen using in vivo microdialysis (Fig. 3.4) (83). We have hypothesized that this decrease in striatal glutamate may be related to the decrease in motor movement that is observed in patients with Parkinson’s disease (83). Following exercise, there is a surprising decrease in striatal extracellular glutamate and that in the 6-OHDA-lesioned rats, there is an additional or additive decrease in extracellular glutamate (Fig. 3.4). However, this is associated with a decrease in the apomorphine-induced contralateral rotations (Fig. 3.2), a finding consistent with a similar decrease in rotations following a lesion of the motor cortex (85). The finding of a further decrease in extracellular glutamate in the 6-OHDA/exercise group is also consistent with a recent report that exercise results in a decrease in the regional cerebral blood flow in the motor cortex and striatum (86). This suggests that as the animal continues to exercise, the corticostriatal pathway requires less activation in order to perform either the same task or it becomes more efficient at carrying out that same locomotor activity. This has also been shown to occur in humans (87, 116). This could result in changes in either the number of corticostriatal synapses, increased efficiency in neurotransmitter release (88, 89), and the induction of long-term potentiation (90), or an increase in the number of post-synaptic glutamate receptors (91).
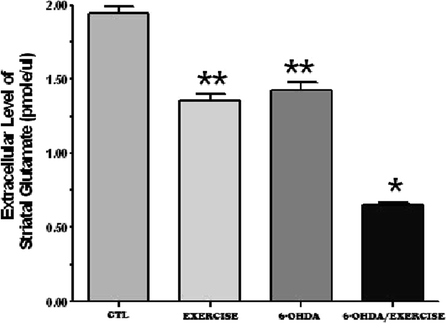
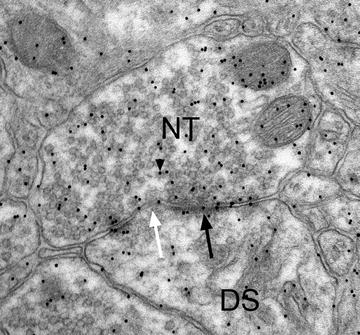
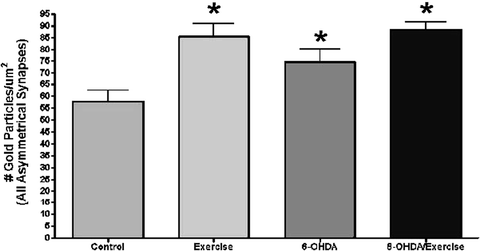
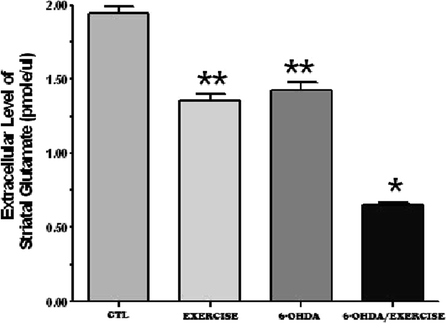
Fig. 3.4.
Exercise decreases the extracellular levels of striatal glutamate. Two months following a 6-OHDA lesion or sham lesion (control), the animals exercised for 1 h/day for 1 month (5 days/week). At the end of the 1-month time period, the basal extracellular levels of glutamate were determined in the dorsolateral striatum. Exercise alone (exercise) resulted in a decrease in striatal glutamate levels, similar to that seen after a 6-OHDA lesion (6-OHDA). The combination of a 6-OHDA lesion plus exercise resulted in a further decrease in striatal extracellular glutamate. * p <0.05 compared to all other groups using an ANOVA, followed by Tukey–Kramer for comparison of multiple means. ** p < 0.05 compared to the control and 6-OHDA/exercise group using an ANOVA, followed by Tukey–Kramer for comparison of multiple means.
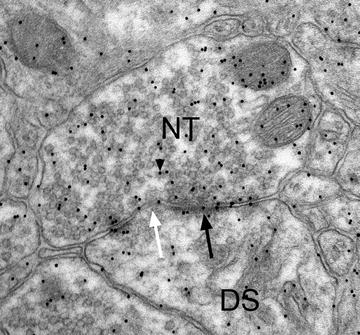
Fig. 3.5.
Nerve terminal (NT) is making an asymmetrical synaptic contact (arrow, pointing to the post-synaptic density) with an underlying dendritic spine (DS). The post-synaptic density is discontinuous or perforated (white arrow). Within the NT are numerous 10-nm gold particles (arrowhead) indicating the location of the neurotransmitter, glutamate.
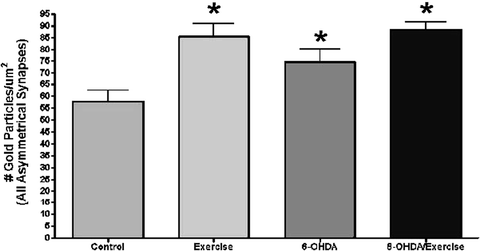
Fig. 3.6.
Exercise the density of glutamate immunogold labeling within nerve terminals making an asymmetrical (excitatory) synaptic contact within the dorsolateral striatum. Two months following a 6-OHDA lesion or sham lesion (control), the animals exercised for 1 h/day for 1 month (5 days/week). At the end of the 1-month time period, the animals were perfused with fixative and the density of glutamate immunogold labeling within identified nerve terminals of the dorsolateral striatum calculated. In all three experimental groups, there was an increase in the density of immunogold labeling compared to the control group. This is inversely correlated to the decrease in extracellular glutamate as determined by in vivo microdialysis (see Fig. 3.4). * p < 0.05 compared to the control group using an ANOVA, followed by Tukey–Kramer for comparison of multiple means.
The procedure used to carry out the nerve terminal glutamate immunogold labeling (Fig. 3.5) is detailed below and is a modification of the procedure previously reported by Phend et al. (92).
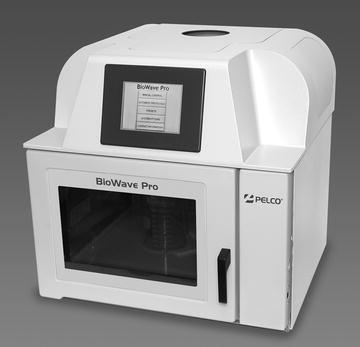
(1)
Animals are first anesthetized and perfused transcardially with the following fixative at room temperature: 2.5% glutaraldehyde/0.5% paraformaldehyde/0.1% picric acid, in 0.1 M HEPES buffer, pH 7.3. The brain is removed and placed in fixative overnight. It is critical that the brain be fixed overnight or the post-embed immunogold procedure will not work. However, do not fix the tissue for more than just overnight.
(2)
The brain is then washed in HEPES buffer several times and the brain can continue to be washed in buffer for up to 2 weeks before being processed for electron microscopy.
(3)
The tissue is cut either with a vibratome (50–300 μm slices) or by hand.
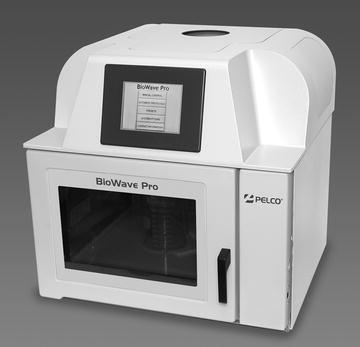
Fig. 3.7.
PELCO BioWave Pro model from Ted Pella, Inc., used for ultrastructural immunolabeling (Cat #36500). Photograph used by permission from Ted Pella, Inc.
Table 3.4
Microwave procedure for tissue processing
PROTOCOL: NORMAL EM TISSUE PROCESSING | |||||||
---|---|---|---|---|---|---|---|
PROGRAM #6 | |||||||
NAME: EM PROCESSING | |||||||
Steps no. | Description | User prompt | Time (h:min:s) | Watts | Temp | Load cooler | Vacuum |
1 | OSMIUM ON | ON | 0:03:00 | 100 | 60 | AUTO | CYCLE |
2 | OSMIUM OFF | OFF | 0:02:00 | 0 | 60 | AUTO | CYCLE |
3 | OSMIUM ON | OFF | 0:03:00 | 100 | 60 | AUTO | CYCLE |
4 | OSMIUM OFF | OFF | 0:02:00 | 0 | 60 | AUTO | CYCLE |
5 | OSMIUM ON | OFF | 0:03:00 | 100 | 60 | AUTO | CYCLE |
6 | RINSE | ON | 0:00:40 | 150 | 60 | AUTO | OFF |
7 | RINSE | ON | 0:00:40 | 150 | 60 | AUTO | OFF |
8 | UA | ON | 0:02:00 | 100 | 60 | AUTO | CYCLE |
9 | UA | OFF | 0:02:00 | 0 | 60 | AUTO | CYCLE |
10 | UA | OFF | 0:02:00 | 100 | 60 | AUTO | CYCLE |
11 | 50% ETOH | ON | 0:00:40 | 150 | 60 | AUTO < div class='tao-gold-member'>
Only gold members can continue reading. Log In or Register a > to continue
![]() Stay updated, free articles. Join our Telegram channel![]() Full access? Get Clinical Tree![]() ![]() ![]() |