Clinical features
Injury type
Gross features
Histological features
Clinical frequency (%)
Chapter
Animal models
Solid Cord Injury
Normal
Demyelination, motor neuron loss
10
Felts et al., ch. 19
None
Laceration
Meningeal disruption, connective tissue scar
Fiber disruption across the lesion, systemic cell infiltration
20
Bates et al., ch. 20
Full or hemi-transection, selective tract suction
Maceration (compression)
Loss of cord topography
Fiber disruption across the lesion
20
Overview this chapter
Aneurysm clip, epi- or sub-dural balloon
Contusion
Fluid-filled cyst, rim of spared tissue surrounding epicenter, no connective tissue scar
Hemorrhage, necrosis, glial scar
50
Methods this chapter
Full or unilateral contusion, MASCIS, OSU, IH or other modified weight-drop devices
The first of these, solid cord injury, is characterized by a cord that grossly appears normal without discoloration, softening or cyst formation. However, upon histological analysis dorsal column demyelination and motor neuron loss can be observed (3). Injuries belonging to this category are rarely observed clinically (10% of patients) and currently there are no animal models in use to study this type of injury. The second category is that of laceration, which is most commonly observed clinically due to penetrating objects or sharp bone fragments (3). Injuries of this type are characterized by disruption of the meninges resulting in widespread systemic cell infiltration and a connective tissue scar formed at the lesion epicenter that often adheres to the overlying dura (2, 3). Animal models of lacerating injury include bilateral or hemitransection of the cord in which the cord is exposed and tissue severed with a sharp object. Alternatively, suction may be applied to selectively ablate motor or sensory tracts (5).
Benefits of laceration models include the ease with which one can target lesion site and size as well as the potential ability with which this model can be used to quantify regeneration techniques following injury (5). This injury type, however, is observed relatively rarely clinically (21% of cases (6)), and as such, laceration models do not reflect the majority of human SCIs. The third category of injury is that of cord maceration or compression. This injury results from massive compression of the cord resulting in large disruption of the spinal cord anatomy such that cord topography is lost (2, 3). Very few fibers are observed crossing the length of the lesion, distinguishing this injury type from that of contusion. Compression injury is modeled in animals using aneurysm clips (7) and epidural (8) or subdural (9) balloons resulting in prolonged disruption of blood flow to cord tissue (5). About 20% of clinical cases fall into the compression category.
The fourth injury category is that of contusion, characterized by contusive injury to the spinal cord resulting in areas of hemorrhage and necrosis that ultimately give rise to a fluid-filled cyst at the lesion epicenter with a rim of spared (although generally dysmyelinated) tissue at the pial surface of the cord (3, 10). The meninges remain intact resulting in less systemic cell infiltrate and a scar surrounding the lesion consisting of virtually no connective tissue components, distinguishing this injury type from laceration injury. The majority of clinical cases of SCI (70%) are contusive-type injuries with some degree of compression, acute or chronic, resulting from fracture/dislocation of the spinal column and subsequent compression of the cord (4, 5, 10). As such, there are many animal models of this type of SCI that have been shown to have pathophysiological elements comparable to those observed in human cases of SCI (5).
1.2 Animal Models of Contusive SCI
Various mechanisms to induce contusion injuries to the spinal cord have been employed for almost a century and have evolved from simple weight-drop techniques to sophisticated computer-controlled devices capable of controlling precise injury parameters. These advances have resulted in animal injury models that have been well characterized, are reproducible, and produce models with a high degree of relevance to human SCI pathophysiology (4). As contusion and compression often both contribute to observed cases of SCI (5), both types of injury models will be described here.
The earliest contusion model was that described by Allen in 1911 in which weights were dropped onto canine spinal cords exposed by laminectomy (11). By dropping weights from different distances gravity is employed to apply differing forces and result in differing compression of the spinal cord. Others later applied this model to make the first assessments of histological changes (12), vasculature impairments (13), myelin breakdown and edema (14) following SCI.
In 1975, force transducers were added to the weight-drop model to quantify the force of impact in primate models (15). Subsequently, canine, feline, and primate models were used to describe progressive tissue damage processes in finer detail as well as the effects of putative therapeutics in SCI (16, 17). Metabolic dysfunction, myelin breakdown, free radical production, respiratory function, ionic shifts, blood–brain-barrier breakdown, cellular immunity, glutamate release and the roles of necrotic and apoptotic cell death were described as reviewed by Young (10). This gave rise to the current understanding of a biphasic injury process in which primary mechanical tissue trauma causes complex, interacting biochemical processes resulting in secondary progressive tissue damage (2, 18).
The elucidation of these delayed secondary injury mechanisms formed the rationale for using therapeutics targeting these processes to limit motor deficit. In 1985, a weight-drop contusion device similar to those described previously was used in a rat model (19, 20). Use of contusion SCI models in the rat now dominates the field of SCI research and has led to quantitative assessments of injury-induced alterations in ascending and descending fiber tracts, neurons and neuronal networks, gliosis, and motor and sensory functional deficits/recovery (10). Indeed the effects of methylprednisolone, the only clinically accepted acute pharmacological treatment of SCI, were evaluated pre-clinically using the contusion model in the rat (21).
1.3 Physical Characteristics of Contusive SCI
All models of contusive SCI essentially involve the application of a force to the spinal cord, leaving the meningeal layers intact (3). The spinal cord is tolerant of acute compression or stretching by 1/3 its normal length with no significant damage if the deforming force is applied slowly. However, prolonged deformations (longer than 20 min) or rapid deformations (past the critical velocity of 0.5–1.0 m/s) result in cell damage and areas of infarct (10, 22). Contusive forces cause tissue to move along the rostral-caudal axis of the spinal cord with the movement being greatest in the middle of the cord and decreasing toward the pial surface and with greater distance from the site of contusion (10). These differential movements account in part for the distribution of tissue damage typically observed following contusive SCI.
Longitudinal movement of tissue shears cells in the gray matter and stretches axons in the white matter (10). Myelinated axons stretch and ultimately break at the nodes of Ranvier when stretching velocities approach 0.5–1.0 m/s, however lower stretching velocities can also result in axonal damage by interfering with membrane channel function, leading to alterations in intracellular ionic concentrations. Large myelinated axons are most vulnerable to contusion, with those at the pial surface most likely to survive (23). This results in a spared rim of tissue surrounding the lesion epicenter. Smaller axons are more likely to be damaged in response to ischemic insults as a result of their smaller surface area to volume ratios (23). However, most cases of SCI include both contusive and compressive components and as such both injury processes are likely to be at work (4, 5). In contusive SCI models, it has been shown that the rate of cord compression correlates linearly with tissue damage and behavioral recovery (10).
1.4 Contusion Impactors and Devices
The weight-drop device employed by Allen in 1911 was composed of weights dropped from differing heights onto the exposed spinal cord, and as such, employed gravity to apply differing forces onto the spinal cord (11). Limitations of early impactor devices included the lack of precise force measurements being applied to the cord, inability to account for displacement of the vertebral column and movement of the animal during impact, and limited range of forces available to the experimenter. Injury parameters have since evolved from gram-centimeter used to describe the height of a weight dropped onto exposed spinal cord to more precise parameters describing the physical nature of the injury such as force applied, tissue compression, impact velocity, and duration (24).
The New York University (NYU) impactor is a weight-drop device designed in 1989 to circumvent some of the limitations of earlier impactor systems (25). Briefly, a 10-g rounded steel rod with a diameter slightly smaller than the vertebral column is threaded onto a rod and placed into a tube positioned above the dorsal aspect of the spinal cord exposed by laminectomy. The weight is then dropped from various vertical heights through the tube directly onto the spinal cord at the mid-thoracic level. The spinal column is supported and suspended to prevent impact energy absorption by the animal’s body mass and to minimize column displacement. Digital optical potentiometers assess the location and timing of the dropped weight providing information about the velocity of the weight at maximum impact as well as biomechanical parameters upon impact (26). These sensors also serve to measure displacement of the spinal column whereupon this extraneous movement is subtracted to give accurate measurements of spinal cord tissue displacement. These features increase the reliability and reproducibility of the device and allow for exclusion of impact severities which fall outside accepted values. This system, however, does not account for rebound of the weight and a second impact from about 10% of the original height occurs, possibly adding significant injury effects (27, 28).
The Ohio State University electromagnetic spinal cord injury device (ESCID) was designed in 1992 and is distinguished from other injury devices in that it utilizes an impactor driven by a solenoid-controlled device as opposed to weights dropped from various heights to initiate injury (24). This device measures cord displacement as well as the force imparted to the tissue and a range of injury severities can be produced by the researcher by specifying tissue displacement values. Rapid contusive injuries (<25 ms) are produced that eliminate rebound to generate a single impact. The ESCID is adaptable to both mice and rats.
The Infinite Horizon (IH) impactor (Precision Systems and Instrumentation, Lexington, KY) is a commercially available impactor system (see Fig. 1). This device differs from the ESCID in that the parameter used to define injury severity is the force applied to the tissue as opposed to tissue displacement (4, 29). The device makes use of a stepping motor that moves the impactor tip vertically with a precision of 1/1,200th of an inch and superfast microcontrollers that sample the force applied at the impactor tip to allow a high precision feedback. This results in a highly accurate injury device and reproducible injury effects.
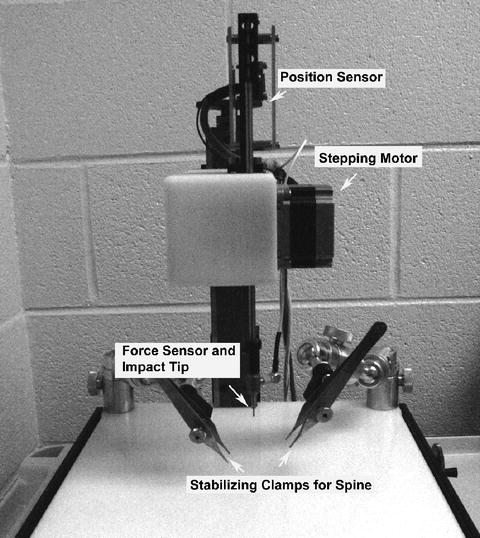
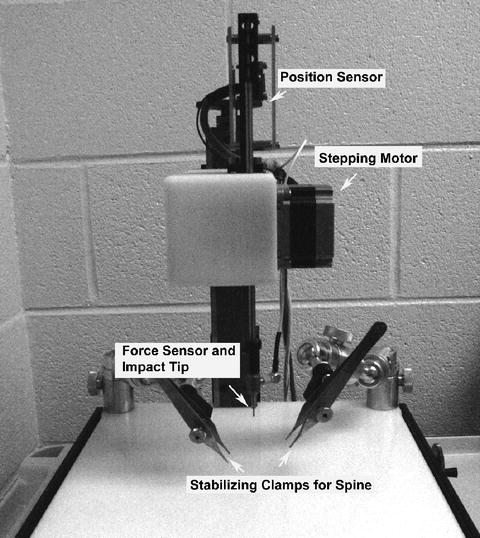
Fig. 1.
Picture of the Infinite Horizons (IH) impactor device. The IH impactor device is used in the protocol described in this chapter. The device comprises an impactor tip which is driven by a stepping motor and controlled by feedback from force and position sensors. The animal is stabilized by clamping Adson micro-forceps onto the spinal column. The spinal cord contusion is induced by impacting the spinal cord with the impact tip. The spinal cord was exposed by a laminectomy at the vertebral segment(s) of interest.
Measurements of applied force and tissue displacement over the course of the impact sequence as well as impactor tip velocity at the time of maximal impact are immediately displayed, thus decreasing error introduced by displacement of the spinal column and allowing the experimenter to assess injury accuracy. Additionally, various compression durations can be implemented in which the impactor tip is held at the preselected force on the surface of the spinal cord for a predetermined time period to model a contusion/compression type injury. As with the ESCID, the IH Impactor eliminates complications caused by rebound of the impactor tip onto the tissue and a wide range of tip diameters allows experimenters to create full contusions in rats and mice as well as rat hemicontusions.
As cord diameter changes with animal age and this parameter can change the effects of impact onto the tissue (10), multiple impactor tip sizes allow a wide age range of animals to be utilized with this device. This model has been characterized in both thoracic and cervical SCI and behavioral as well as recovery data are in agreement with those described using the NYU impactor (1, 29, 30). The main advantages of the IH impactor include the increased range of injury severities produced, the ability to produce contusion/compression-type injuries, the flexibility with which the experimenter can change impactor tip sizes to suit their needs, and the fact that it is commercially available.
1.5 Cervical SCI
The early models of contusive SCI were performed in the mid-thoracic region of the spinal cord resulting in hindlimb deficits while allowing the animal to ambulate to some extent via preserved function of the forelimbs (10). The mid-thoracic injury model has been used throughout the last century and has increased our understanding of SCI pathophysiology and progression substantially and led to characterization of widely used quantitative analyses of hindlimb motor and sensory function.
However, over 50% of clinical cases of SCI occur in the cervical region (31). Indeed, clinical cases of SCI rarely occur at the mid-thoracic region for several reasons (3, 32). First, the cervical spinal column is highly flexible and as such the vertebrae are relatively unstable and at greater risk for fracture than at the mid-thoracic region where the vertebrae are stabilized by the ribcage (22). Second, a smaller epidural space in the cervical region increases the likelihood that fracture of the vertebrae will result in impingement upon the spinal cord (22, 24).
Although over 50% of clinical cases of SCI occur in the mid-cervical region, most research is performed using a mid-thoracic injury model (31). Several key anatomical differences between these two regions, however, question the application of results obtained from mid-thoracic models to a predominantly cervical SCI clinical population (31). The first of these is the relative gray–white matter distribution. The thoracic cord is composed almost entirely of white matter, with the gray matter innervating the small intercostal musculature (31). Alternatively, the cervical cord has the largest gray matter area by volume of all spinal cord regions, as lower motor neuron pools innervating the upper extremity are housed in this region (33). As such, deficits evaluated in animal models of mid-thoracic SCI are primarily attributable to white matter damage, whereas deficits following cervical injury include both white and gray matter pathology (30, 31). As the physical properties, metabolic demands, and cell types of white and gray matter differ, it stands to reason that the pathophysiology following SCI may also differ between these two cord regions (24).
Secondly, target innervations of these regions differ. As mentioned previously, functional deficits resulting from mid-thoracic injury are typically quantified through hindlimb motor function and hind-fore limb coordination which is based on hindlimb locomotor abilities that are attributable to white matter sparing. In contrast, the cervical region controls the upper extremities in both rats and humans. For example, carbanocyanine tracing studies have demonstrated that cervical motor neuron topography is conserved between rats and humans and that rats are capable of complex prehensile movements of the forepaw comparable to those seen in the human hand (33, 34). Consequently, quantitative assessments of forelimb function have recently been characterized in rodent models of cervical SCI (30–32, 35). These assessments appear to be capable of demonstrating more subtle changes in motor function than the classical hindlimb function assessments, which may reveal subtle effects of potential therapeutics that may be missed if employed in a mid-thoracic injury model.
As cervical spinal cord lesions result in fore- and hindlimb dysfunction, hemicontusion models have been developed to ensure mobility and health of the animal (36). The motor and sensory deficits observed following this type of injury reflect those observed in the clinical example of Brown-Sequard syndrome, a unilateral injury of the spinal cord (37). The corticospinal tract (CST) is the main descending motor tract. Since the majority of CST fibers decussate in the medulla (pyramidal decussation) and therefore above the level of the lesion, the C5 hemicontusion injury results in impairments in motor function in the ipsilateral forelimb and less pronounced deficits in the ipsilateral hindlimb. No motor deficits are observed contralateral to the injury (30, 32, 34).
The spinothalamic tract, the main ascending tract responsible for pain sensation, decussates at the level it enters the spinal cord. Therefore, a C5 hemicontusion injury results in contralateral neuropathic pain-like behaviors in dermatomes below the level of the lesion and ipsilateral in the dermatome corresponding to the lesion site. Taken together, these studies indicate that a cervical hemicontusion injury model in rats appears to correlate well with motor and sensory deficits observed in human cases and can be a powerful tool in the quest to discover novel interventions for the treatment of SCI.
2 Materials
2.1 Tools for Pre-surgical Preparation of Rats (See Note 1)
Electric fur trimmer or other method for removal of fur
Petroleum ophthalmic ointment (e.g., PURALUBE ointment)
Povidone Iodine 5% solution (e.g., BETADINE solution)
Antimicrobial Skin Cleanser (e.g., 2% chlorhexidine scrub, CHLORHEXIDERM solution)
2.2 Surgical Tools
Ear Punch (Fine Science Tools 24210-02) (see Note 2)< div class='tao-gold-member'>Only gold members can continue reading. Log In or Register a > to continue
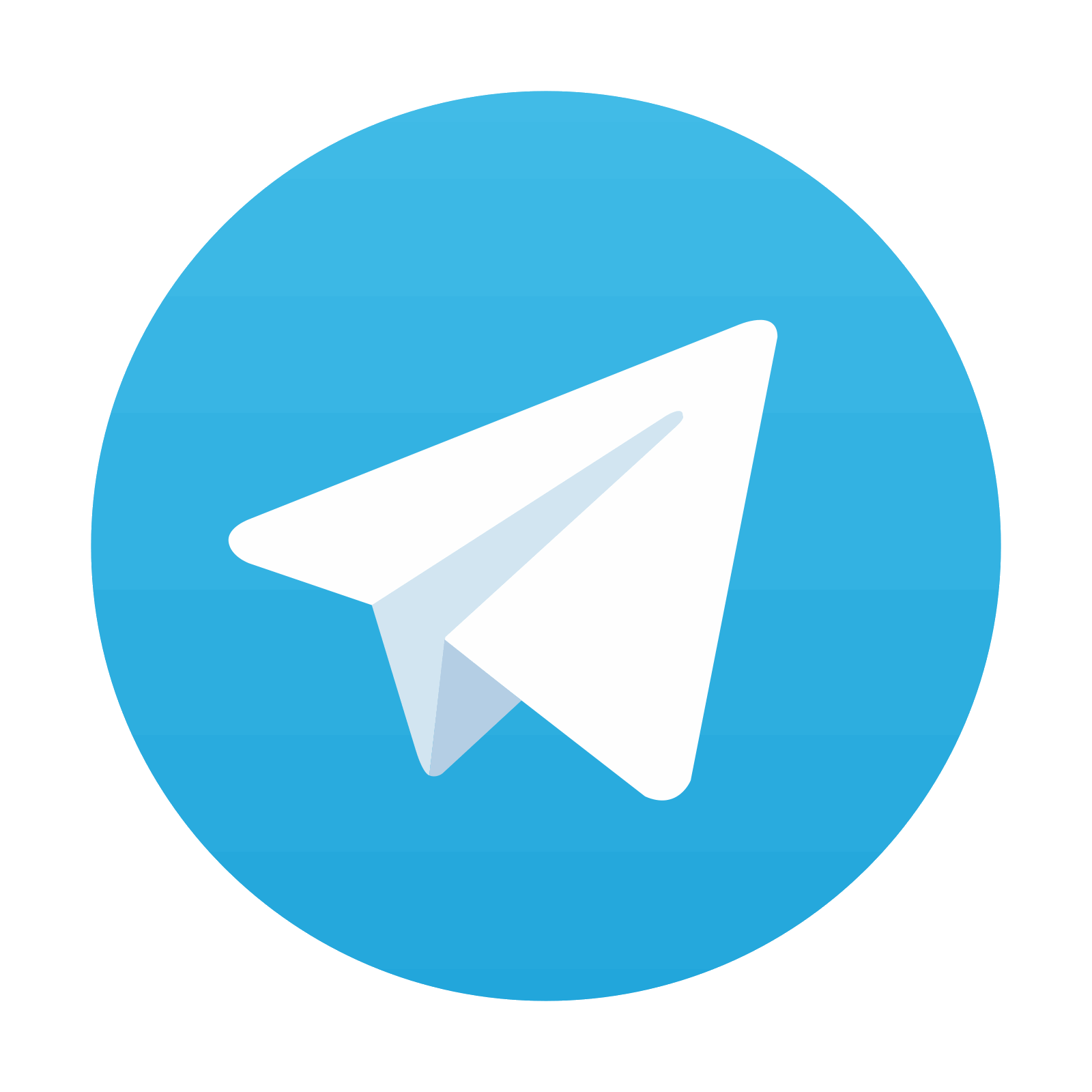
Stay updated, free articles. Join our Telegram channel
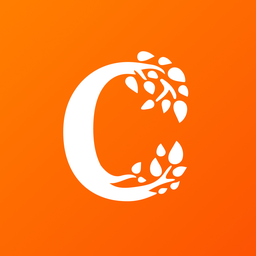
Full access? Get Clinical Tree
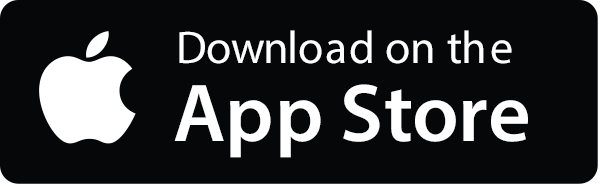
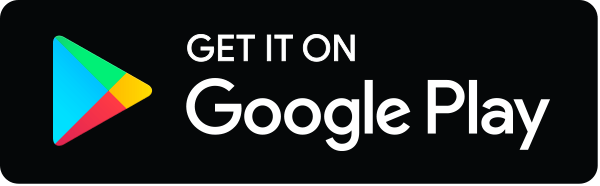