Chapter 26 Cerebrospinal Fluid II. FUNCTIONS OF CEREBROSPINAL FLUID III. CSF FORMATION, CIRCULATION, AND ABSORPTION A. Anatomy of Brain-Fluid Interfaces IV. CELLULAR COMPOSITION OF NORMAL CSF A. Total Erythrocyte and Nucleated Cell Count V. BIOCHEMICAL CONSTITUENTS OF NORMAL CSF B. Proteins in the Cerebrospinal Fluid G. Concentration Gradient along the Neuraxis VI. CSF COLLECTION AND ANALYTICAL TECHNIQUES B. Physical Examination: Clarity, Color, and Viscosity VII. GENERAL CHARACTERISTICS OF CSF IN DISEASE A. Physical Characteristics: Clarity, Color, and Viscosity VIII. CHARACTERISTICS OF CSF ASSOCIATED WITH SPECIFIC DISEASES F. Malformations of Neural Structures G. Metabolic/Nutritional Disorders L. Trauma/Compression of Neural Tissue The analysis of cerebrospinal fluid (CSF) has been described as the central nervous system (CNS) equivalent of the complete blood count (Jamison and Lumsden, 1988), and the analogy is appropriate. CSF analysis is a general index of neurological health and often provides evidence of the presence of disease. Similar to a complete blood count, CSF analysis has reasonable sensitivity but low specificity. The possible alterations of CSF are relatively limited compared to the varieties of neurological diseases that exist (particularly if the analysis is restricted to total cell counts and total protein determination). Additionally, the type and degree of CSF abnormality seem to be related as much to the location of disease as to the cause or the severity of lesion; meningeal and paraventricular diseases generally produce greater abnormalities than deep parenchymal diseases. Previous therapy may affect the type, degree, and duration of CSF (Jamison and Lumsden, 1988) abnormalities as well. The CSF abnormalities identified are also dependent on the CSF collection site with respect to lesion location (Thomson et al., 1989, 1990). Lastly, the CSF of animals with neurological disease is not always abnormal (Tipold et al., 1995). Only occasionally does CSF analysis provide a specific diagnosis (Kjeldsberg and Knight, 1993)—for example, if infectious agents (bacteria or fungi) or neoplastic cells are observed. In most situations, the chief utility of CSF analysis is to assist in the diagnostic process by excluding the likelihood of certain disease processes being present. As is the case with all tests of relatively low specificity, examination of CSF is most useful when the results are correlated with the history, clinical findings, imaging studies, and ancillary laboratory studies. As stated by Fankhauser (1962), “It is futile to make a diagnosis based solely on the CSF findings and particularly on single alterations of it. Only the entire picture of all findings linked with the other clinical symptoms is of value in reaching a diagnosis.” Cerebrospinal fluid has four major functions: (1) physical support of neural structures, (2) excretion and “sink” action, (3) intracerebral transport, and (4) control of the chemical environment of the central nervous system. Cerebrospinal fluid provides a “water jacket” of physical support and buoyancy. When suspended in CSF, a 1500-gm brain weighs only about 50 gm. The CSF is also protective because its volume changes reciprocally with changes in the volume of intracranial contents, particularly blood. Thus, the CSF protects the brain from changes in arterial and central venous pressure associated with posture, respiration, and exertion. Acute or chronic pathological changes in intracranial contents can also be accommodated, to a point, by changes in the CSF volume (Fishman, 1992; Milhorat, 1987; Rosenberg, 1990). The direct transfer of brain metabolites into the CSF provides excretory function. This capacity is particularly important because the brain lacks a lymphatic system. The lymphatic function of the CSF is also manifested in the removal of large proteins and cells, such as bacteria or blood cells, by bulk CSF absorption (see Section II.D). The “sink” action of the CSF arises from the restricted access of water-soluble substances to the CSF and the low concentration of these solutes in the CSF. Therefore, solutes entering the brain, as well as those synthesized by the brain, diffuse freely from the brain interstitial fluid into the CSF. Removal may then occur by bulk CSF absorption or, in some cases, by transport across the choroid plexus into the capillaries (Davson and Segal, 1996; Fishman, 1992; Milhorat, 1987; Rosenberg, 1990). Because CSF bathes and irrigates the brain, including those regions known to participate in endocrine functions, the suggestion has been made that CSF may serve as a vehicle for intracerebral transport of biologically active substances. For example, hormone releasing factors, formed in the hypothalamus and discharged into the CSF of the third ventricle, may be carried in the CSF to their effective sites in the median eminence. The CSF may also be the vehicle for intracerebral transport of opiates and other neuroactive substances (Davson and Segal, 1996; Fishman, 1992; Milhorat, 1987; Rosenberg, 1990). An essential function of CSF is the provision and maintenance of an appropriate chemical environment for neural tissue. Anatomically, the interstitial fluid of the central nervous system and the CSF are in continuity (see Section II.A); therefore, the chemical composition of the CSF reflects and affects the cellular environment. The composition of the CSF (and the interstitial fluid) is controlled by cells forming the interfaces, or barriers, between the “body” and the neural tissue. These semipermeable interfaces, the blood-brain barrier, the blood-CSF barrier, and the CSF-brain barrier, control the production and absorption of CSF and provide a fluid environment that is relatively stable despite changes in the composition of blood (Davson and Segal, 1996; Fishman, 1992; Milhorat, 1987; Rosenberg, 1990). The brain (and the spinal cord) as an organ is isolated in many ways from the body and the systemic circulation. This isolation is accomplished anatomically by several interfaces between brain tissue and systemic fluids (Table 26-1). At these interfaces, selective carriers and ion pumps transport electrolytes and essential nutrients and thereby control the brain’s microenvironment. A substantial portion of this control is achieved through the formation, circulation, and absorption of CSF at these brain-fluid interfaces (Davson and Segal, 1996; Fishman, 1992; Milhorat, 1987; Rosenberg, 1990). The important blood-brain (and blood-spinal cord) interface is formed by the endothelial cells of the intraparenchymal capillaries. In most areas of the brain and spinal cord, the capillary endothelium differs from that of other body tissues in the following ways: (1) the absence of fenestrae, (2) the presence of tight junctions between adjacent cells, (3) a lower number of pinocytotic pits and vesicles, (4) a higher number of mitochondria, and (5) closely applied, perivascular, astrocytic foot processes. These features result in the capillary endothelium being a selective barrier—the blood-brain barrier—that regulates the entry, and probably the exit, of biologically important substances and aids in the maintenance of a precise, stable environment for the neural tissues (Davson and Segal, 1996; Fishman, 1992; Milhorat, 1987; Rosenberg, 1990). The epithelial cells of the circumventricular organs form one part of the blood-CSF interface. The circumventricular organs, which include the four choroid plexuses, the median eminence, the neural lobe of the hypophysis, and other specialized areas, border the brain ventricles and are involved with specific secretory activities that appear to require a direct contact with plasma. The capillaries within these organs are fenestrated, similar to capillaries in other organs of the body. Overlying each of the organs are specialized epithelial cells joined by intercellular tight junctions at their apical (ventricular) borders. These epithelial cells also are characterized by an abundance of intracellular organelles and lysosomes. These organelles are probably an important aspect of the barrier and secretory functions of these cells (Davson and Segal, 1996; Fishman, 1992; Milhorat, 1987; Rosenberg, 1990). The choroid plexuses are the major source of CSF. They are formed by evaginations of the ependyma and the pial blood vessels into the ventricles, and they consist of a single row of cuboidal, specialized epithelial cells thrown into villi around a core of blood vessels and connective tissue. The apical (ventricular) surface of the epithelial cells has a brush border of microvilli with occasional cilia. The basal and lateral cell surfaces have numerous infoldings. Overall, the structure of these cells resembles other epithelia specialized for fluid transport, such as proximal renal tubular epithelium (Davson and Segal, 1996; Fishman, 1992; Milhorat, 1987; Rosenberg, 1990). Autonomic nerve terminals have also been identified in the choroid plexus, but their function is unclear (Fishman, 1992; Nilsson et al., 1992). The second part of the blood-CSF interface is formed by the arachnoid membrane at the arachnoid villi. These villi are microscopic evaginations of the arachnoid membrane into the lumen of the dural sinuses. The barrier function of these arachnoid cells is demonstrated by their tight junctions. Their transport function is indicated by giant intracellular vacuoles, some of which have both basal and apical openings, and pinocytotic vesicles. The sinus surface of a villus is covered by sinus endothelium (Milhorat, 1987; Rosenberg, 1990). Endothelium-lined channels may link directly with the subarachnoid space (Bell, 1995; Davson and Segal, 1996). Arachnoid villi are not limited to intracranial venous sinuses but also are present at the spinal nerve roots penetrating into the spinal veins (Bell, 1995; Milhorat, 1987). The extensive CSF-brain (and spinal cord) interface consists of the ependyma within the cavities of the central nervous system and the pia mater covering the central nervous system. These two layers are each composed of a single layer of cells joined by gap junctions. The ependyma and the pia mater are not important permeability barriers; the CSF (ventricular and subarachnoid) and the brain interstitial fluid are directly continuous (Davson and Segal, 1996; Milhorat, 1987). Cerebrospinal fluid is formed principally by the choroid plexuses, with a smaller amount formed extrachoroidally (Davson and Segal, 1996; Milhorat, 1987). Choroidal formation involves two processes that occur in series; first is filtration across the choroidal capillary wall, and second is secretion by the choroidal epithelium. Within the choroid plexus, hydrostatic pressure of the choroidal capillaries initiates the transfer of water and ions to the interstitial fluid and then to the choroidal epithelium. Water and ions are then transferred into the ventricles either by (1) intracellular movement across the epithelial membranes, or (2) intercellular movement across the apical tight junctions between epithelial cells. Both of these processes probably depend on ion pumps. Secretion of CSF results from the active transport of sodium, which is dependent on the membrane-bound, sodium-potassium activated ATPase present at the apical (ventricular) surface of the choroidal epithelium (Davson and Segal, 1996; Rosenberg, 1990). The presence of autonomic nerve terminals in the choroid plexus suggests a neural control of CSF secretion. However, the functional role of this innervation in normal and pathological conditions is unknown (Fishman, 1992; Nilsson et al., 1992). Spurred primarily by clinical evidence that excision of the choroid plexus did not benefit human patients with hydrocephalus, experimental evidence now supports the existence of an extrachoroidal source of CSF. The diffusion of brain interstitial fluid across the ependyma or pia mater is the apparent source of this extrachoroidal CSF component. Formation of the interstitial fluid is thought to occur by active transport processes (secretion) at the cerebral capillaries, but an alternative theory proposes passive permeability of the capillary endothelium and active transport by the surrounding astrocytes (Milhorat, 1987; Rosenberg, 1990). The relative contributions of choroidal and extrachoroidal sources to CSF in normal and pathological conditions are not certain. Some investigators report the choroid plexus to be the major if not the sole source of CSF; whereas others conclude that at least one-third of newly formed CSF is extrachoroidal (Davson and Segal, 1996; Milhorat, 1987). TABLE 26-2 Rate of CSF Formation in Various Speciesa Modified from Davson and Segal (1996). aEstimated by ventriculo-cisternal perfusion. Regardless of the amount of extrachoroidal formation, the rate of CSF formation is closely correlated to the weight of the choroid plexus and varies among species (Table 26-2) (Cserr, 1971; Welch, 1975). Increases and decreases in formation rate have been achieved experimentally, but the general tendency is for the formation rate to remain relatively constant. The formation rate directly parallels the rate of sodium exchange, which is linked to the bicarbonate ion. The enzyme carbonic anhydrase plays an important role because it provides the bicarbonate. Inhibition of carbonic anhydrase slows (but does not abolish) sodium, bicarbonate, and chloride flow, resulting in a reduction of CSF secretion (Maren, 1992). Several drugs and conditions inhibit CSF production (Table 26-3), but their clinical utility is limited either by their time frame of action or toxicity (Davson and Segal, 1996; Pollay, 1992; Rosenberg, 1990). Moderate variations in intracranial pressure probably do not affect CSF formation. However, studies of chronically hydrocephalic animals have shown a reduction of CSF formation with increasing intraventricular pressure. The secretion process may also be affected by chronically increased intracranial pressure (Fishman, 1992). Cerebrospinal fluid flows in bulk from sites of production to sites of absorption. Fluid formed in the lateral ventricles flows through the paired interventricular foramina (foramen of Monro) into the third ventricle, then through the mesencephalic aqueduct (aqueduct of Sylvius) into the fourth ventricle. The majority of CSF exits from the fourth ventricle into the subarachnoid space; a small amount may enter the central canal of the spinal cord. In people, CSF enters the subarachnoid space through the lateral apertures (foramina of Luschka) and the median aperture (foramen of Magendie) of the fourth ventricle. Animals below the anthropoid apes do not have a median aperture (Fankhauser, 1962; Fletcher, 1993). Cerebrospinal fluid has also been shown to flow from the spinal subarachnoid space into the spinal perivascular spaces, across the interstitial space, then into the central canal (Stoodley et al., 1996). Mechanisms for propelling the CSF along its route probably include (1) the continuous outpouring of newly formed ventricular fluid, (2) the ciliary action of the ventricular ependyma, (3) respiratory and vascular pulsations, and (4) the pressure gradient across the arachnoid villi (Milhorat, 1987). Absorption of CSF occurs by bulk absorption of the fluid and by absorption or exchange of individual constituents of the fluid (i.e., ions, proteins, and drugs). Bulk absorption occurs directly into the venous system and depends primarily on the CSF hydrostatic pressure; as the pressure rises, the absorption rate increases (Davson and Segal, 1996). If intracranial pressure falls below a critical point, bulk absorption decreases, a homeostatic response to stabilize the intracranial pressure and the CSF volume. The primary site of bulk absorption, at least in people, is the arachnoid villi that project into the dural sinuses. Two other routes are through lymphatic channels in the dura and through the perineural sheaths of cranial nerves (particularly the olfactory nerves) and spinal nerves. Perineural absorption may be through arachnoid villi projecting into perineural veins, lymphatics, or connective tissue (Davson and Segal, 1996; Milhorat, 1987). The importance of these various absorption routes varies with the species (Bell, 1995). Absorption through the arachnoid villi occurs trans-cellularly through micropinocytotic vesicles and giant intracellular vesicles, but it may also occur through endothelium-lined, intercellular clefts. The mechanisms appear to vary among species (Bell, 1995). Absorption is unidirectional from the CSF into the venous blood—the villi act like one-way valves. The basis for the valve-like mechanism appears to be transport by the giant vesicles (see Section II.A.2). Particles ranging in size from colloidal gold (0.2 μm) to erythrocytes (7.5 μm) can be transported across the villi. In disease conditions, accumulations of larger size particles (e.g., protein molecules, erythrocytes, leukocytes) within the villi may impair absorption leading to hydrocephalus (Fishman, 1992; Milhorat, 1987). The choroid plexus also has an absorptive function, acting on specific substances in the CSF rather than by bulk fluid absorption. A variety of compounds are actively transported from the CSF, in a fashion reminiscent of the proximal renal tubule. Solutes may also be cleared from the CSF by diffusion into adjacent brain cells or capillaries (Fishman, 1992; Milhorat, 1987). Cerebrospinal fluid normally does not contain erythrocytes (Chrisman, 1992; Cook and DeNicola, 1988; Rand et al., 1990b; Wilson and Stevens, 1977). Erythrocytes in a CSF sample are most commonly iatrogenic, because of trauma associated with the needle placement. However, CSF erythrocytes may also originate from pathological hemorrhage. The normal nucleated cell count of CSF in domestic animals is in Table 26-4. The most widely accepted reference ranges for the numbers of leukocytes in the CSF of dogs and cats is 0 to 5 cells/μL (de Lahunta, 1983; Oliver and Lorenz, 1993) to 0 to 8 cells/μL (Duncan, 1994). However, these ranges are too broad in our experience and other studies confirm this (Jamison and Lumsden, 1988). Jamison examined 50 clinically and histopathologically normal dogs and derived cerebellomedullary CSF reference limits of 0 to 2 cells/μL (Jamison and Lumsden, 1988). In fact, all except one of these dogs had counts of 0 to 1 cells/μL (personal communication). Bailey and Higgins examined 31 dogs that were clinically and histopathologically normal. For cerebellomedullary CSF, the mean nucleated cell count was 1.45 cells/μL with the 95% confidence intervals 1.04 to 1.86, and the observed range 0 to 4 cells/μL. Twenty-six of 31 dogs had counts between 0 to 2 cells/μL. They also found that lumbar CSF had a significantly lower nucleated cell count, with a mean of 0.55 cells/μL, a 95% confidence interval of 0.22 to 0.88 and an observed range of 0 to 4 cells/μL, although 30/31 dogs had counts of 0 to 2 cells/μL (Bailey and Higgins, 1985). In our opinion, a normal nucleated count for cerebellomedullary CSF in dogs is 0 to 2 cells/μL, with 3 cells/μL being questionably abnormal and 4 cells/μL definitely abnormal. Rand and colleagues (1990b) derived reference limits for cerebellomedullary CSF from 33 cats that were clinically and histopathologically normal. The samples did not have blood contamination. The mean ± 1 SD for the white blood cell count was 0.1 ± 0.4 with an observed range of 0 to 2 cells/μL. Thirty of 33 cats had counts of 0 cells/μL. Three cells or more per microliter is therefore abnormal in feline cerebellomedullary CSF. Excellent morphological descriptions of the cell types normally found in the CSF of domestic animals can be found elsewhere (Cook and DeNicola, 1988; Jamison and Lumsden, 1988; Rand et al., 1990b). Normal CSF consists of varying proportions of small lymphocytes and monocytes. The proportions are species and age dependent (Kjeldsberg and Knight, 1993). In dogs, monocytic type cells predominate (Jamison and Lumsden, 1988), although there is individual variation. In cats (Jamison and Lumsden, 1988; Rand et al., 1990b), (unreactive) macrophages also predominate, with a mean of 87%, whereas small lymphocytes have a mean of 9%. This same trend is observed in horses, with 73.6% monocytes (macrophages) and 26.2% lymphocytes (Furr and Bender, 1994). However, small lymphocytes predominate in cattle (Welles et al., 1992) and llamas (Welles et al., 1994). In the literature published before 1975 that focused on humans, any neutrophils present in the CSF were thought to be indicative of disease (Kjeldsberg and Knight, 1993). However, with the advent of techniques for concentrating CSF specimens, such as cytocentrifugation, it became clear that a very small number of neutrophils may be found in normal human CSF (Fishman, 1992; Kjeldsberg and Knight, 1993). Similar observations have been made in many veterinary species, and rare neutrophils may be a normal finding in the CSF of all domestic species. Eosinophils are not present in normal CSF, although a single cell is occasionally seen on cytocentrifuge slides in animals with normal total nucleated cell counts. Large foamy activated macrophages or phagocytes are not seen in normal CSF (Christopher et al., 1988; Fishman, 1992), and their presence is nonspecific evidence of an inflammatory disorder. Plasma cells are not seen in normal CSF (Fishman, 1992; Kjeldsberg and Knight, 1993; Pelc et al., 1981). Their presence indicates underlying inflammatory disease. In people, plasma cells are seen particularly in acute viral disease and various chronic inflammatory conditions including tuberculous meningitis, syphilis, multiple sclerosis, and the Guillain-Barré syndrome (Kjeldsberg and Knight, 1993; Pelc et al., 1981). In animals, plasma cells have been observed in various conditions including distemper (Vandevelde and Spano, 1977), other viral meningitis (Bichsel et al., 1984a; Vandevelde and Spano, 1977), rabies (Green et al., 1992), granulomatous meningoencephalomyelitis (Bailey and Higgins, 1986a; Vandevelde and Spano, 1977), neoplasia, and abscesses (W. Vernau, personal observations). Therefore, although they are abnormal, no specificity is associated with their presence in CSF. Similarly, reactive lymphocytes are not found in normal CSF, but their presence has no specificity. They can be seen in active or resolving infectious disease, immune mediated diseases, and neoplasia (Cook and DeNicola, 1988). Cells other than leukocytes can be seen in both normal and abnormal CSF. Cells lining the leptomeninges, choroid plexus cells, and ependymal cells can be seen as single cells or, more often, as small papillary clusters or sheets. Cytologically, choroid plexus cells and ependymal cells are indistinguishable (Cook and DeNicola, 1988; Garma-Avina, 2004; Kjeldsberg and Knight, 1993). The majority of lining cells seen in normal CSF is choroid plexus cells (Kjeldsberg and Knight, 1993). Excellent descriptions and illustrations of these cell types can be found elsewhere (Cook and DeNicola, 1988; Garma-Avina, 2004; Kjeldsberg and Knight, 1993; Rand et al., 1990b). Chondrocytes are occasionally observed in CSF sampled by lumbar puncture, likely resulting from the spinal needle puncturing the intervertebral disk (Bigner and Jonston, 1981). Squamous cells can be observed in CSF and may be due to skin contamination or an underlying pathological process such as epidermoid cysts (Kornegay and Gorgacz, 1982) or metastatic carcinomas. Bone marrow cells (immature hematopoietic precursors) have been described in the CSF of people (Kjeldsberg and Knight, 1993) and dogs (Christopher, 1992). In people, bone marrow cells in the CSF are usually associated with lumbar puncture, usually in infants or in patients with vertebral bone abnormalities that create difficulties during the sampling process. The cells are present because of sampling from the vertebral body or articular process bone marrow. Christopher (1992) observed hematopoietic cells in the lumbar CSF of two dogs and speculated that it was due either to marrow penetration or to dural extramedullary hematopoiesis (Christopher, 1992). Extramedullary hematopoiesis was observed in the choroid plexus of five dogs that did not have underlying hematological abnormalities or the presence of extramedullary hematopoiesis elsewhere (Bienzle et al., 1995). Although the CSF was normal in these dogs and hematopoietic cells were not observed, this site could provide another potential source for the presence of these cells in CSF. Metastatic myeloid leukemia could conceivably produce similar findings, but peripheral blood and marrow examination would clarify the origin of the cells in question. Neurons, astrocytes, glial cells, and neural tissue may be observed in the CSF of people (Bigner and Jonston, 1981) and also in cerebellomedullary cisternal samples associated with traumatic CSF taps in animals (Fallin et al., 1996). White matter in CSF is more common in lumbar versus cisternal CSF samples in dogs, most likely because of the sampling method,1 although the presence of underlying malacia is another potential cause (Mesher et al., 1996). White matter in the CSF is not correlated with a negative prognosis when compared to dogs without white matter in the CSF.1 Because CSF is a product of plasma filtration and membrane secretion, its composition is different from plasma. In general, CSF is a clear, colorless, nearly acellular, low protein fluid. Various ions, enzymes, and other substances are also found in normal CSF. In health, the CSF composition is maintained relatively constant by the various membrane interfaces, although some fluctuations occur with fluctuations in plasma composition. The chemical composition of the CSF of various animal species is summarized in Tables 26-5 through 26-8. These values should serve only as a guide; normal values must be established for individual laboratories. In people and animals, differences in CSF appearance and composition exist between neonates and adults. Human neonatal CSF is usually xanthochromic, probably because of a greater protein and bilirubin content than adult CSF. Glucose content is also increased, more closely approximating the blood glucose level. Many of these differences (e.g., protein content) are attributed to immaturity of the blood-brain barrier. Immaturity of the blood-brain barrier may be due to an increased number of fenestrae in the brain capillaries or inadequate closure of their endothelial tight junctions. Other factors that may contribute to age differences in CSF composition are the integrity of the blood-CSF barrier, the rate of CSF secretion and efficiency of absorption, the volume of the extracellular space of the brain, and the lipid-solubility of the substances (Davson and Segal, 1996; Fishman, 1992). Protein also decreases with age in foals and puppies (Furr and Bender, 1994; Meeks et al., 1994; Rossdale et al., 1982). In contrast, two studies of calves found that CSF protein increased with age (Binkhorst, 1982; St. Jean et al., 1995). Foals also had xanthochromia and a higher CSF glucose and creatine kinase level than adults (Furr and Bender, 1994; Rossdale et al., 1982). The white blood count (WBC) decreased with age in puppies and calves (Binkhorst, 1982; Meeks et al., 1994). Studies done in prenatal, neonatal, and adult laboratory animals (including rats, rabbits, pigs, sheep, cats, dogs, and monkeys) and people have shown that, in general, the CSF/plasma concentration ratios (RCSF) of Na+, Mg 2+, and Cl– increase with age. The RCSF of K+, HCO3–, and urea decrease. In some instances, however (e.g., Cl– and K+), changing plasma levels of these substances contribute to the change in the RCSF. The RCSF of total protein, as well as the individual proteins, decreases with age. The decreasing concentration of proteins in the CSF compared to plasma protein is an indication of the maturation of the blood-brain and blood-CSF barriers. In rats, the RCSF of amino acids also falls quickly with age, although large individual variations exist. Taurine, for example, has a higher level in the adult than the newborn. This fact, as well as the species-specific transport of some proteins (e.g., albumin) into the CSF, indicates a special mechanism of transport based on factors other than molecular weight (Davson and Segal, 1996). Proteins identified in the CSF are given in Tables 26-9 and 26-10. In general, the concentration of a CSF protein is inversely related to its molecular weight. If the blood-brain barrier is normal, serum proteins with a molecular weight greater than 160,000 daltons are largely excluded. However, Felgenhaur (1974) reported CSF:serum protein distribution ratios to be better correlated with the hydrodynamic radii than with the molecular weight of the proteins. Almost all the proteins normally present in CSF are derived from the serum. The exceptions are transthyretin (prealbumin) and transferrin, which are also synthesized by the choroid plexus, and beta and gamma trace proteins, tau protein (tau fraction, modified transferrin), glial fibrillary acidic protein, and myelin basic protein, which appear to be synthesized intrathecally (Thompson, 1988). With electrophoretic techniques, protein in the CSF can be separated into prealbumin, albumin, and alpha, beta, and gamma globulins. The major protein in CSF is albumin, which is synthesized only in the liver. The limited entry of albumin into the CSF is dependent on the blood-brain/CSF barrier to macromolecules. When total CSF protein increases, the albumin concentration increases disproportionately. This phenomenon illustrates the role of molecular size in determining the distribution of serum proteins into the CSF (Felgenhauer, 1974). Immunoelectrophoresis can separate the alpha and beta globulins into several proteins (Table 26.9). The origin of tau protein (beta2 transferrin) is uncertain. This protein may be modified serum transferrin (beta1 transferrin) or it may be a unique protein, “tau protein,” in the CSF (Fishman, 1992). In veterinary and human medicine, no correlation has been made between changes in the concentrations of these globulins and specific neurological disease (Fishman, 1992; Sorjonen et al., 1991). Thus, their measurement has limited clinical use at this time. Because of the changes found in association with multiple sclerosis and other inflammatory diseases, the gamma globulins have received a great deal of attention. Electrophoretic techniques define the gamma globulins as a heterogeneous group of proteins with migrations at similar rates (see Table 26.9). The gamma globulin fraction contains immunoglobulins. Immunological assays identify three major immunoglobulins in normal CSF: IgG, IgM, and IgA. Minute amounts of other immunoglobulins have also been detected in normal CSF (Fishman, 1992; Kjeldsberg and Knight, 1993). TABLE 26-9 Cerebrospinal Fluid Proteins Identified by Electrophoresis (Top Row) and Immunoelectrophoresis (Underlying Columns) The major immunoglobulin in normal CSF is IgG, which normally originates from the serum. An increased level of CSF gamma globulin is reported in a number of inflammatory central nervous system disorders. In disease conditions, gamma globulin may enter the CSF through dysfunctional blood-brain/CSF barriers, or it may be synthesized intrathecally by cells that have migrated into the brain or CSF and are participating in the disease process (Fishman, 1992; Kjeldsberg and Knight, 1993). Cerebrospinal fluid IgM and IgA also originate normally from the serum. However, in certain diseases, particularly inflammatory diseases, these immunoglobulins are produced within the central nervous system as well (Fishman, 1992; Kjeldsberg and Knight, 1993). IgM is ontogenetically and phylogenetically the most primitive immunoglobulin and is therefore detected at an earlier stage of the general immune response of the body. IgM is also the first immunoglobulin to return to normal when the offending antigen disappears. The characteristics of IgM and IgA participation in the intrathecal immune response still need to be resolved, however (Felgenhauer, 1982; Tipold and Jaggy, 1994). Many other proteins have been identified in CSF including myelin basic protein, S-100 protein, C-reactive protein, interferon, embryonic proteins, fibronectin, and glial fibrillary acidic protein. In general, the CSF concentrations of these proteins may be altered by a number of neurological disease processes. The utility of assaying these proteins in clinical veterinary or human medicine has yet to be established (Fishman, 1992; Kjeldsberg and Knight, 1993). CSF glucose is derived solely from the plasma by facilitated diffusion. The concentration of CSF glucose depends on the blood glucose concentration, the rate of glucose transport into the CSF, and the metabolic rate of the central nervous system. The normal CSF glucose level is about 60% to 80% of the blood glucose concentration, reflecting at least in part the high metabolic rate of the central nervous system. Equilibration with plasma glucose requires about 1 to 2 h; thus, ideally, plasma glucose should be determined about 1 h before CSF aspiration and analysis. In people, a glucose gradient exists along the neuraxis; the concentration decreases from ventricular to lumbar fluid (Fishman, 1992; Kjeldsberg and Knight, 1993). In people, a CSF:serum glucose ratio less than 0.4 to 0.5 is abnormal and associated with bacterial and fungal infections, as well as metastasis to the leptomeninges (Deisenhammer et al., 2006). CSF glucose and serum CSF:glucose ratio is not routinely used in veterinary medicine, possibly because of the lack of specificity and availability of more specific tests in most instances. Numerous enzymes have been assayed in the CSF of animals (see Tables 26.5 to 26.8) (Jackson et al., 1996; Lobert et al., 2003; Rand et al., 1990a; Wilson, 1977) and people (Banik, 1983). These enzymes have three possible sources: (1) blood, (2) neural tissue or neural tumors, and (3) cells within the CSF (Fishman, 1992; Kjeldsberg and Knight, 1993). The blood enzyme levels are usually higher than the CSF levels. Unfortunately, many studies of CSF levels in disease fail to report the concurrent blood level and a measure of blood-brain/CSF barrier integrity. However, studies of CSF creatine kinase (CK) in dogs and horses did not find a relationship between WBC counts, serum CK, or CSF total protein and CSF CK (Furr and Tyler, 1990; Jackson et al., 1996). Regarding correlation of CSF red blood count (RBC) with CSF CK, one study reported a significant correlation (Indrieri et al., 1980), whereas another study did not find a statistical association between the two parameters (Jackson et al., 1996). CSF lactate is independent of blood glucose, and it may be measured in people and animals (Deisenhammer et al., 2006; Lobert et al., 2003). In people, blood:CSF lactate ratio may be elevated with mitochondrial disease and correlates inversely with the blood:CSF glucose ratio. To date, none of the enzyme assays is sufficiently sensitive or specific to warrant routine use in clinical practice (Fishman, 1992; Indrieri et al., 1980; Jackson et al., 1996; Kjeldsberg and Knight, 1993; Rand et al., 1994a). Because they are produced by neurons, neurotransmitters and their metabolites have been extensively studied in people for their potential use as markers of neuronal activity and neurological and psychiatric disease (Davis, 1990). The concentrations of several neurotransmitters (e.g., γ-aminobutyric acid [GABA], glutamate, aspartate and dopamine) and their metabolites (e.g., 5-hydroxyindolacetic acid, homovanillic acid, and dihydroxyphenylacetic acid) have been measured in the CSF from various sites in dogs, sheep, goats, cattle, and horses (Bardon and Ruckebusch, 1984; Ellenberger et al., 2004; Faull et al., 1982; Holt et al., 2002; Loscher and Schwartz-Porsche, 1986; Podell and Hadjiconstantinou, 1997; Ruckebusch and Costes, 1988; Ruckebusch and Sutra, 1984; Sisk et al., 1990; Vaughn et al., 1988a, 1989). Some metabolite concentrations have a gradient along the neuraxis (Ruckebusch and Costes, 1988; Ruckebusch and Sutra, 1984; Vaughn et al., 1988b; Vaughn and Smyth, 1989), and some are age-related (Ruckebusch and Costes, 1988; Smyth et al., 1994; Vaughn and Smyth, 1989). Despite intense interest, more research is needed to verify the clinical utility of assay of these substances in the CSF (Fishman, 1992; Kjeldsberg and Knight, 1993). Many other substances have been measured in CSF in experimental and clinical situations. These include electrolytes, gases, organic and amino acids, ammonia, urea, creatinine, prostaglandins, cytokines, and hormones. Assay of these substances is not particularly helpful in the diagnosis of neurological disease in people because the substances are not generally associated with specific disease (Fishman, 1992; Kjeldsberg and Knight, 1993). Some substances, such as S-100B, a calcium-binding protein, have been used as a marker protein in people with brain injury, but its utility in veterinary medicine is unproven (Shimada et al., 2005). The usefulness of these substances in veterinary medicine has yet to be established. In cats (Hochwald et al., 1969), dogs (Bailey and Higgins, 1985; Vaughn et al., 1988b), horses (Andrews et al., 1990a; Vaughn and Smyth, 1989), rhesus macaques (Smith and Lackner, 1993), and people (Davson and Segal, 1996; Fishman, 1992), the total protein concentration increases along the neuraxis from rostral to caudal. For example, in people the total protein concentration of ventricular, cerebellomedullary cistern, and lumbar subarachnoid fluid is about 26, 32, and 42 mg/dl, respectively (Weisner and Bernhardt, 1978). Total protein, albumin, and globulin content of cerebellomedullary cistern and lumbar subarachnoid CSF for dogs, cats, and horses is given in Tables 26.5, 26.6, and 26.7, respectively. The concentration of the albumin and globulin fractions also increases from ventricular to lumbar fluid. The increased protein content may be the result of a greater permeability of the spinal blood-CSF barrier than of the ventricular barrier to albumin (Fishman, 1992), additions of protein from adjacent nervous tissue (e.g., IgG from lymphocytes located in or near the CSF pathway (Weisner and Bernhardt, 1978), progressive equilibration of CSF with plasma through the capillary walls (Weisner and Bernhardt, 1978), and low flow rates of lumbar CSF (Davson and Segal, 1996). A study of healthy dogs also identified a small but significant gradient for the CSF WBCs; lumbar fluid contained significantly fewer cells than cerebellomedullary fluid (Bailey and Higgins, 1985). Another study did not find a difference in WBC counts between fluids from the two sites (Vaughn et al., 1988b). However, 4 of the 10 dogs in this study had CSF total WBC counts >3/μl, and none of the dogs was necropsied to verify its health. Therefore, some of these dogs may have had subclinical neurological disease, disguising a small cellular gradient. The small number of WBCs in normal fluid may make a cellular gradient more of a theoretical issue than a practical issue, however. If a cellular gradient exists, it may be due to fewer cells entering the lumbar CSF than the cerebellomedullary CSF, a greater rate of cell lysis in the lumbar CSF, a greater migration rate of WBCs from lumbar CSF back into the blood, or loss of WBCs that entered the CSF rostrally and lysed as CSF circulated to the caudal subarachnoid space. A gradient has also been reported for CSF neurotransmitter metabolites in the dog (Vaughn et al., 1988b) and the horse (Vaughn and Smyth, 1989). In each species, the neurotransmitter metabolite content of cerebellomedullary CSF was greater than that of lumbar subarachnoid CSF. This gradient probably reflects the major source of the neurotransmitter (brain) and transport of the metabolite from the CSF into the blood along the spinal axis (Vaughn et al., 1988a). Specific details about the collection of CSF from the various species are covered in many excellent articles and textbooks (Boogerd and Peters, 1986; Brewer, 1983, 1987, de Lahunta, 1983; Fowler, 1989; Holbrook and White, 1992; Kornegay, 1981; Mayhew, 1989) and will not be covered here except for the authors’ preferred technique for collection from the cerebellomedullary cistern of dogs and cats (discussed later). Considerations that apply regardless of species are sterility, use of a specialized spinal needle, and collection from animals with increased intracranial pressure. To prevent iatrogenic central nervous system infection, sterility during the collection procedure is essential. A generous area around the puncture site should be clipped and surgically prepared. Preparation of too small an area can lead to contamination if any difficulty in palpating landmarks or entering the subarachnoid site is encountered. Additionally, the use of a fenestrated drape is highly recommended. Spinal puncture is contraindicated in an area of severe pyoderma/furunculosis or cellulitis. A needle with a stylet (spinal needle) should be used to prevent implantation of a plug of epidermis in the subarachnoid space that not only could lead to infection but also could seed an epidermoid tumor. Replacement of the stylet upon withdrawal is controversial, either preventing or causing entrapment and severance or dislocation of nerve root filaments (Fishman, 1992). Collection of CSF from animals with increased intracranial pressure may result in brain herniation. Appropriate anesthetic agents, hyperventilation, and mannitol (to treat intracranial hypertension) may decrease the probability of herniation. Use of the smallest gauge needle possible may also help prevent herniation by decreasing CSF leakage through the puncture hole in the meninges. Only the minimal amount of CSF necessary to perform the desired tests should be withdrawn. Brain herniation can occur following lumbar taps as well as cerebellomedullary cistern taps. Cerebellomedullary puncture should be done under general anesthesia. In most instances, lumbar puncture can be done with sedation and local anesthesia. Therefore, if general anesthesia is contraindicated, a lumbar puncture should be done. The choice of collection site is influenced by the species and breed of animal, the location of the neurological lesion, and anesthetic considerations. The size of some animals may make lumbar subarachnoid puncture difficult, if not impossible. However, cerebellomedullary puncture usually can be accomplished even in large or obese animals. Because of differences in anatomy, the type or breed influences the exact site for lumbar puncture in the dog; L4-5 is recommended for large breed, nonchondrodystrophic dogs (e.g., German shepherd dogs), whereas L5 to 6 is recommended for small, chondrodystrophic dogs (e.g., dachshunds) (Morgan et al., 1987). The puncture site chosen should be as close to the lesion as possible without penetrating the lesion, or the site should be caudal to the lesion. In animals with spinal disease, cerebellomedullary fluid is abnormal more frequently with cervical disease than it is with thoracolumbar disease, but overall lumbar fluid is abnormal more often than cerebellomedullary fluid. With intracranial disease, CSF from both sites is usually abnormal, perhaps because both sites are caudal to the lesion (Scott, 1992; Thomson et al., 1989, 1990). Occasionally, CSF is collected from both sites. Although the order of collection (cerebellomedullary or lumbar CSF collected first) appears not to influence significantly the analytical results (Bailey and Higgins, 1985), aspiration from the relatively small lumbar subarachnoid space is easier if the CSF pressure has not just been lowered by cerebellomedullary CSF collection. The authors’ preferred technique for CSF collection from the cerebellomedullary cistern is to utilize the palpable bony landmarks that are the closest to the puncture site. These structures are the vertebral arch of C1 and the external occipital protuberance. After anesthetic induction and intubation, the animal is placed in right lateral recumbency, and padding is placed under the neck to align the dorsal cervical and cranial midline parallel to the tabletop. The assistant is instructed to tuck in the animal’s chin (flex the neck) and push the external occipital protuberance toward the operator. This procedure flexes the atlantooccipital joint and maximizes the space between the occipital bone and C1. Asking the assistant to simply flex the neck seems to produce flexion of the midcervical area more than the atlantooccipital area. The clinician faces the dorsal aspect of the patient’s neck, kneeling on a pad. The external occipital protuberance, the C2 spinous process, and the C1 vertebral arch are palpated. The latter structure is located by rolling a fingertip off the cranial edge of the C2 spinous process and palpating firmly, feeling for a transverse bony ridge (the C1 vertebral arch). The C1 vertebral arch can usually be palpated, and if so, the puncture is made on the midline just in front of the fingertip palpating the vertebral arch. If C1 is not palpable, the distance between the cranial edge of the C2 spinous process and the occipital protuberance is noted, and the puncture is made on the midline about one-third of that distance cranial to the cranial edge of the C2 spinous process. In rare cases, neither C1 nor C2 can be palpated. In this situation, the lateral edge of each C1 transverse process is palpated and a triangle from each edge to the occipital protuberance is constructed visually. The puncture is made on the midline in the center of that triangle. The needle should be advanced slowly and the stylet removed regularly. A “pop” may be palpated when the dura mater is punctured with the needle. The clinician should hold the spinal needle with one hand (to hold it steady) and remove the stylet with the other hand. The CSF should be allowed to drip out of the spinal needle into a tube. A volume of at least 0.5 mLs should be collected for a full CSF analysis (partial analysis may be done with smaller volumes). Larger volumes may be collected for other tests such as culture and sensitivity, polymerase chain reaction for infectious agents, antigen/antibody testing, immunophenotyping, and clonality assessment. To collect CSF for culture and sensitivity testing, aspirate CSF directly from the spinal needle hub using a needle and syringe. After collection, the CSF is examined visually and the color, clarity, and viscosity are recorded. Normal CSF is clear and colorless and has essentially the same viscosity as water. For accurate assessment, the CSF can be compared to the same amount of distilled water in the same type of container. The containers can be held against a white, typewritten page to judge color and clarity, and gently shaken to assess viscosity. If the CSF appears abnormal, the color and clarity of the supernatant after centrifugation should be noted. Collection of CSF in a plastic or silicon coated glass tube is preferred because monocytes will adhere to glass and can activate in the process (Fishman, 1992). This can result in erroneous cell counts and also alter morphology. In practical terms, this is of little consequence in those specimens that are rapidly processed, but it becomes important as the delay between collection and processing increases. A complete cytological examination includes both a total and differential cell count, as well as thorough morphological assessment. A differential and thorough morphological assessment should be done routinely, even on those samples that have cell counts within normal limits. In our experience, very low cell counts alone cannot be used as an indicator of normality. In one study utilizing cytocentrifugation, about 25% of canine CSF samples with cell counts in the normal range had abnormalities in cell type or morphology (Christopher et al., 1988). Abnormalities included the presence of phagocytic macrophages, increased percentage of neutrophils in the differential, and the presence of reactive lymphocytes and plasma cells. Malignant cells have been observed in samples with normal nucleated cell counts (Bichsel et al., 1984b; Grevel and Machus, 1990). CSF samples should be processed as soon as possible after collection. Cells degenerate quickly in CSF (Chrisman, 1992; Fishman, 1992; Fry et al., 2006; Kjeldsberg and Knight, 1993; Steele et al., 1986), likely secondary to the CSF hypotonicity and very low protein content (in nonpathological specimens). Proteins and lipids tend to have a membrane stabilizing effect (Fry et al., 2006; Steele et al., 1986). A multitude of veterinary references state that CSF must be processed within 30 min of collection (Chrisman, 1983; Cook and DeNicola, 1988; Oliver and Lorenz, 1993; Thomson et al., 1990). However, these references do not cite scientific data to support this statement. One study systematically evaluated the effects of time, initial composition and stabilizing agents on the results of abnormal (TNCC ≥ 5 cells/ul) canine CSF evaluation (Fry et al., 2006). Statistically significant changes (p = <0.05) in the total nucleated cell count were not noted at any time point (0, 2 h, 4 h, 8 h, 12 h, 24 h, and 48 h) in unaltered (refrigerated) CSF, CSF with added fetal calf serum, or CSF with added hetastarch. However, differential cell percentages deteriorated in a time dependent fashion and macrophages were the most labile cell type in this study with their differential percentage being significantly decreased by 2 h. Concurrently, the percentage of unrecognizable cells was significantly increased at 2 h. At 12 and 24 h, the percentages of lymphocytes and neutrophils, respectively, were significantly decreased. Samples with a higher protein concentration (≥50 mg/dl) were less susceptible to deterioration than those with a lower protein concentration (<50 mg,dl). The addition of fetal calf serum or hetastarch improved the stability of the CSF. Ultimately, the authors supported the contention that CSF should be analyzed as soon as possible post collection but that delays of 4 to 8 h were unlikely to alter the overall clinical interpretation (Fry et al., 2006). If the protein concentration is > 50 mg/dL, the analysis may be delayed up to 12 h without altering the overall clinical interpretation as the mean percentage of unrecognizable cells was only 6% at this time point (versus 33% in samples with protein concentration <50 mg/dl) (Fry et al., 2006). Several reports recommend altering CSF processing when it is not analyzed within 1 h of collection (Bienzle et al., 2000; Fry et al., 2006). If there is a delay in processing, CSF samples should be divided into two aliquots. The unaltered aliquot should be submitted for TNCC and protein concentration. The second aliquot should be treated by the addition of either 20% fetal calf serum or 10% autologous serum, and the differential cell counts and morphology should be assessed on the second altered aliquot. In a study of feline CSF, there was excellent correlation between the total numbers of cells on the slides and the differential cell count between sediment slides processed immediately and those preserved with fetal bovine serum (200 μl of CSF and 200 μl of fetal bovine serum) and cytocentrifuged 2 to 4 h later (Rand et al., 1990b). There have also been several human studies performed on the effects of time and temperature on CSF (Kjeldsberg and Knight, 1993; Steele et al., 1986; Stokes et al., 1975). Interestingly, in these studies, and in contrast to the above study assessing canine CSF, neutrophils and not large mononuclear cells were the most labile cell type. Refrigeration at 4°C markedly reduced the rate of lysis of all cell types in the human CSF studies. Therefore, the recommendation that analysis be performed within 30 min is reasonable, but it is predicated by the conditions that the sample is exposed to. Refrigeration obviously slows lysis, likely long enough for transport to reference laboratories in some instances. Addition of protein to the sample helps preserve cells and therefore attenuates the temporal effects involved in transport of samples to more remote facilities. Electronic cell or particle counters are typically not sensitive enough to be used for enumeration of cells in CSF. The level of background counts with these counters is frequently in excess of the counts present in the majority of CSF samples that are analyzed. Therefore, cells are usually counted with a standard hemacytometer chamber with Neubauer ruling (Brobst, 1989; Cook and DeNicola, 1988; Jamison and Lumsden, 1988). The chamber is charged with undiluted fluid. Ideally, the cells are allowed to settle for 10 min in a humidified environment. This allows all the cells to be visible in the same plane of focus. The cells in the nine largest squares on both sides of the chamber are counted (18 squares in total) and the result multiplied by 0.55 to obtain the number of cells per microliter. Alternatively, the cells in nine large squares are counted and the number multiplied by 1.1 to determine the count per microliter. To the untrained observer, unstained leukocytes and erythrocytes may be difficult to differentiate. Leukocytes are larger, and the presence of nuclei gives them a more granular appearance than erythrocytes. With experience, nuclear morphology can often be appreciated (Cook and DeNicola, 1988). The cytoplasmic border is usually slightly irregular. In contrast, erythrocytes are usually smaller, smooth, and refractile, although they may become crenated upon standing (Jamison and Lumsden, 1988). Differentiating nucleated cells and erythrocytes in a hemacytometer chamber can be expedited by staining with New Methylene Blue before counting (Fry et al., 2006). This latter technique can be used without significant dilutional effects. A laser based cell counter and dedicated software are used to count and differentiate cells in human CSF (Aune et al., 2004; Mahieu et al., 2004). This technique has the advantage of markedly superior precision and accuracy. The same methodology has been used to assess canine CSF (Ruotsalo et al., 2005). Although there was good correlation between the leukocyte and erythrocyte concentrations when compared with standard hemacytometer methods, the current software algorithms were not suitable for determining an accurate differential count in canine CSF. Additionally, it is likely that the cost and logistics of this methodology will preclude routine use in veterinary medicine. There are a variety of methods to facilitate cytological examination of CSF (Barrett and King, 1976; Ducos et al., 1979; Grevel, 1991; Hansen et al., 1974; Jamison and Lumsden, 1988; Kolmel, 1977; Roszel, 1972; Sornas, 1967; Steinberg and Vandevelde, 1974; Woodruff, 1973). There is controversy as to which method is optimal, and all have their strengths and weaknesses. Methods include simple centrifugation, sedimentation, and variations thereof; membrane filtration; and cytocentrifugation (Jamison and Lumsden, 1988). Consult these references for specific methodological details. Simple centrifugation usually produces slides that are unsatisfactory for cytological examination. The chief advantage of membrane filtration techniques is excellent cellular recovery with yields approaching 90% to 100% (Barrett and King, 1976). However, the methodology is laborious and time consuming, the cellular morphology relatively poor, many cells are partly hidden in the filter substance, which itself stains variably, and the technique requires specialized, nonroutine staining techniques that most veterinary clinical pathologists do not have experience or expertise in interpreting. For these reasons, they are not recommended. Cytocentrifugation (Hansen et al., 1974; Woodruff, 1973) is the method of choice in both human (Fishman, 1992; Kjeldsberg and Knight, 1993) and veterinary medicine (Christopher et al., 1988; Jamison and Lumsden, 1988). It is rapid, simple, and produces slides with good cytological detail. The technique is enhanced by the addition of protein to the CSF sample before centrifugation, which helps to preserve cell morphology. Conditions of cytocentrifugation vary from laboratory to laboratory. We prefer the method described by Rand and colleagues (Rand et al., 1990b). The disadvantages of cytocentrifugation are the expense of the instrument and the relatively low cell yield. In one comparative study (Barrett and King, 1976), the following cell yields were determined: millipore filtration 81 ± 3% (SEM), nucleopore filtration 69 ± 3%, and cytocentrifugation 11 ± 1%. The Sornas method of centrifugation results in a cell yield, after staining, of approximately 20% (Sornas, 1967). The sedimentation technique of Sayk, modified by Kölmel (Grevel, 1991; Grevel and Machus, 1990; Kolmel, 1977), results in a yield of approximately 30% (Kolmel, 1977), although this can be increased to almost 90% if a membrane filter is substituted for direct sedimentation onto a slide. We have some experience with the Kölmel apparatus and technique and have found the cell morphology to be at least as good as cytocentrifugation with an apparently superior cell yield, although this needs to be confirmed with controlled comparative studies. Therefore, most studies suggest that sedimentation techniques result in greater cell yields than does cytocentrifugation. However, there is at least one study that found the yield of cytocentrifugation to be marginally higher than sedimentation (Ducos et al., 1979). Standard Romanowsky stains are recommended for staining of slides. They provide good cellular detail on air-dried CSF preparations and are familiar to most observers. These stains include the Wright’s and Wright–Giemsa staining methods as well as a variety of rapid staining methods including Diff-quik and Camco-quik (Jamison and Lumsden, 1988). In people, the value of cytological diagnosis of CSF can be improved if morphological studies are appropriately supplemented by immunocytochemistry (Kjeldsberg and Knight, 1993). Immunophenotypic studies of cytocentrifuge slides are useful in the differential diagnosis of leukemia, lymphosarcoma, primary brain tumors, and metastatic tumors (Bigner, 1992; Bigner and Jonston, 1981; Jorda et al., 1998; Kjeldsberg and Knight, 1993; Tosaka et al., 2001). Few veterinary studies document CSF immunocytochemistry. In dogs, immunophenotyping is useful in the diagnosis of lymphoma and infiltrative leukemia2 and histiocytic sarcoma, both postmortem (Zimmerman et al., 2006) and antemortem.3 Panels of monoclonal antibodies are typically used for the immunocytochemical assessment of CSF. The greatest limitation is therefore the volume and cellularity of the specimen available for the marker studies. Undifferentiated tumor panels frequently include leukocyte common antigen and cytokeratin antibodies. These can be helpful in distinguishing single carcinoma cells from lymphocytes or monocytes (Bigner, 1992; Bigner and Jonston, 1981; Kjeldsberg and Knight, 1993). Glial fibrillary acidic protein has proven to be helpful in distinguishing a glial origin, but there are currently no specific markers to distinguish primary brain tumors. Immunocytochemistry can be used also to characterize the lymphocyte subpopulations present in CSF. Seventy-five to 95% of the lymphocytes found in normal human CSF are T cells, with a mean of approximately 85% (Kjeldsberg and Knight, 1993). Within the population of T cells, T-helper cells predominate and account for up to 88% of T cells. Alterations of these percentages have been shown to have significant associations with disease in people (Kjeldsberg and Knight, 1993). Similar studies assessing CSF lymphocyte subset alterations in disease are lacking in domestic animals but may be useful. Lymphocyte subset distribution has been assessed in the brains of dogs with different types of diseases (Tipold et al., 1999). T cells predominated in viral encephalitides, whereas B cells predominated in bacterial and protozoal diseases and in steroid responsive meningitis-arteritis (Tipold et al., 1999). However, it has not been determined if similar changes are reflected in the CSF. Lymphocyte subset distribution has been assessed in normal dogs and horses (Duque et al., 2002; Furr et al., 2001; Tipold et al., 1998) Similar to people, T lymphocytes predominate in canine CSF but are present as a lower percentage than in people, accounting for approximately 50% to 60% of lymphocytes (Duque et al., 2002; Tipold et al., 1998). However, there appears to be much individual variation that may complicate use of this type of assessment clinically (Tipold et al., 1998). Horses appear to be more similar to people, with T cells accounting for approximately 80% of lymphocytes in the CSF (Furr et al., 2001). However, in contrast to people, CD8 + T cells in normal equine CSF may constitute a greater subset of T cells, accounting for approximately 30% of CSF T cells in one study (Furr et al., 2001). In people, most central nervous system lymphomas are B cell in origin; immunocytochemistry assessing immunoglobulin light chain expression can be used to document monoclonality (Bigner, 1992; Bigner and Jonston, 1981). This is strong evidence (but not definitive proof) of malignancy. This assessment cannot be made in most domestic animals because of the marked light chain skewing that exists normally in these species (Arun et al., 1996: Butler, 1998, #387). The B cell origin of the lymphocytes can also be confirmed with demonstration of immunoglobulin light chain expression. For patients with T cell lymphomas, marker studies can be more difficult to interpret as T cells predominate in normal and inflammatory CSF (Kjeldsberg and Knight, 1993). If there is systemic involvement, then comparison with the peripheral phenotype is useful to confirm presence in the CSF. Immunocytochemistry has also been utilized to detect infectious agents such as cytomegalovirus and mycobacterium tuberculosis in human patients (Stark et al., 1993; Sumi et al., 2002) and distemper virus in dogs (Abate et al., 1998). Polymerase chain reaction (PCR) technology is a powerful adjunct to routine cytological assessment of CSF that may increase both the sensitivity and specificity of diagnosis. Because PCR exponentially increases in vitro the number of original DNA copies to a final number dependent on the number of cycles programmed, it is uniquely suited to the low volumes and small cell numbers frequently found in CSF samples. In people, one of the most useful applications of PCR methodology is the confirmation of malignancy and detection of minimal residual disease in lymphomatous meningitis (Rhodes et al., 1996). This is accomplished via detection of clonal immunoglobulin or T cell receptor gene rearrangements and the detection of clone specific rearrangements, respectively. However, the exquisite sensitivity may result in false positive results because of either contamination or very low initial numbers of cells producing an artifactual clonal band. Tumor specific quantitative reverse transcriptase PCR (qRT-PCR) has been used for the sensitive detection of (neoplastic) neuroblastoma cells in the CSF of a human patient (Rosanda et al., 2006). Other applications in people include detection of a wide variety of infectious agents, such as toxoplasma, borrelia, tuberculosis, human immunodeficiency virus, rabies virus, herpes simplex virus, and various amebas (Christen et al., 1995; Guffond et al., 1994; Lin et al., 1995; Novati et al., 1994; Qvarnstrom et al., 2006). In the majority of these studies, PCR results in a more rapid diagnosis with superior sensitivity and specificity when compared to standard culture and serological diagnostic techniques (Deisenhammer et al., 2006). In veterinary medicine, PCR is used to detect several infectious agents in CSF samples, such as bacteria (listeria monocytogenes and Streptococcus equi), protozoa (sarcocystis neurona, toxoplasma gondii, neospora caninum), and viruses (canine distemper virus, West Nile virus, and equine herpesvirus-1) (Amude et al., 2006a, 2006b; Cannon et al., 2006; Fenger, 1994; Finno et al., 2006; Frisk et al., 1999; Goehring et al., 2006; Kim et al., 2006; Peters et al., 1995; Schatzberg et al., 2003; Stiles et al., 1996). Some agents such as listeria, encysted neospora or toxoplasma bradyzoites in the CNS parenchyma may not gain access to the meningoventricular system. This may result in negative CSF PCR results in confirmed positive cases (Peters et al., 1995). A combination of diagnostic information (clinical information, CSF assessment, serology, PCR, biopsy, and immunohistochemistry) is the most practical way to make a clinical diagnosis, rather than the use of a single test result, such as PCR (Schatzberg et al., 2003). PCR assays for the detection of clonal immunoglobulin or T-cell receptor gene rearrangements in dogs and cats have now been developed (Burnett et al., 2003; Moore et al., 2005; Vernau and Moore, 1999; Werner et al., 2005). These assays have been used for the confirmation of malignancy in the CSF of dogs with suspected CNS lymphoma (W. Vernau, unpublished data). Recently, qRT-PCR was used to assess the cytokine profiles present in the CSF of horses with different neurological disorders (Pusterla et al., 2006b). Some differences were noted between the different types of diseases but significant overlap of values also occurred. Further developmental work in conjunction with additional prospective studies will be required before the true utility of PCR based CSF diagnostics can be accurately assessed in domestic animals. An increase in the concentration of CSF total protein was recognized as an indicator of neurological disease soon after the introduction of lumbar puncture in human medicine. A number of tests were developed to assess qualitative changes in CSF protein, such as Lange’s colloidal gold test, the Nonne-Appelt test, the Pandy test, and others. These qualitative tests have largely been replaced by quantitative methods. Urinary dipsticks have been used to determine CSF protein concentration, but false negative and false positive test results occur using this methodology, which preclude recommendation for routine use (Behr et al., 2003; Jacobs et al., 1990). Techniques for quantitative measurement of CSF total protein include turbidimetric methods, biuret procedures, and Lowry’s method. The accuracy of these methods in many clinical laboratories is no better than ± 5% (Fishman, 1992). Dye binding microprotein assays such as Coomassie Brilliant Blue, Ponceau S red, and Pyrogallol red (Marshall and Williams, 2000; Pesce and Strande, 1973) are more accurate and are now the methods of choice for measurement of CSF protein concentration. Total CSF protein values are reported in numerous articles and vary noticeably with the methodology and the laboratory performing the assay. Therefore, clinicians must use laboratory-specific normal values when assessing CSF protein concentration. A number of techniques for fractionation of CSF proteins have been developed. These include electrophoresis using paper or cellulose acetate, agar, agarose, polyacrylamide, and starch gels. Immunoelectrophoresis, electroimmunodiffusion, radioimmunoassay, isoelectric focusing, and high-resolution protein electrophoresis are more recent techniques (Behr et al., 2006; Fishman, 1992; Kjeldsberg and Knight, 1993). Because of the normally low protein content, most of these methods require concentration of the CSF, which can create technical artifacts in the measured protein content. Techniques that do not require CSF concentration, such as electroimmunodiffusion, are therefore advantageous (Fishman, 1992). Because albumin is synthesized only extrathecally, increased CSF albumin indicates damage to the blood-brain/CSF barriers, intrathecal hemorrhage, or a traumatic CSF tap. In these conditions, albumin will leak into the CSF in general proportion to its serum concentration. Therefore, in the absence of intrathecal hemorrhage (pathological or iatrogenic), the ratio of CSF albumin to serum albumin can be used as an indicator of barrier dysfunction (Link and Tibbling, 1977; Tibbling et al., 1977). This ratio is also called the albumin index (a.k.a. albumin quota, albumin quotient) and is calculated as follows (Kjeldsberg and Knight, 1993): Values above the normal range indicate increased barrier permeability. The use of this index is potentially limited, however, because the large variability of CSF albumin in normal animals (at least in dogs and horses) (Andrews et al., 1990a, 1994; Bichsel et al., 1984b; Krakowka et al., 1981) results in a large variability in the values for this index (Davson and Segal, 1996). In people, the albumin index is age dependent, being highest in newborns, and lowest in childhood. The albumin index increases with age (Deisenhammer et al., 2006). The identification of intrathecal production of immunoglobulin is helpful in the diagnosis of neurological disease. Immunoglobulin G is the dominant CSF immunoglobulin. However, the IgG content of CSF is not a particularly useful measurement by itself because the IgG present in CSF may be of serum-origin (via a dysfunctional blood-brain/CSF barrier, intrathecal hemorrhage, or traumatic puncture) or intrathecally produced (as in various neural diseases). Varied opinions exist regarding the best way to calculate the contribution of IgG from each source (Thompson, 1988; Trotter, 1989). To determine the probable origin of CSF IgG, it can be related mathematically to a protein of purely extrathecal origin. Because albumin is synthesized entirely extrathecally, it is the preferred comparison protein and is the most widely used (Fishman, 1992). Transferrin and alpha2 macroglobulin have also been recommended because of their extrathecal origin (Schliep and Felgenhauer, 1974). The simplest formula for correction of the CSF IgG level for extrathecal “contamination” (Zimmerman et al., 2006), and thereby demonstration of intrathecal IgG synthesis, is the IgG/albumin index (Link and Tibbling, 1977; Tibbling et al., 1977). This index is calculated using the CSF and serum concentrations of albumin and IgG as follows (Kjeldsberg and Knight, 1993): The denominator of this index (CSF albumin/serum albumin) is the albumin index. Because albumin is synthesized only extrathecally, the albumin index assesses the amount of albumin crossing the blood-brain/CSF barriers and therefore is a measure of barrier integrity. Blood contamination of the CSF with as little as 0.2% serum (equivalent to about 5000 to 10,000 RBC/μl) by a traumatic puncture falsely elevates the IgG index in people (Peter and Tourtellotte, 1986). Also, the IgG index loses reliability when CSF protein levels are less than 25 mg/dl or greater than 150 mg/dl (Boerman et al., 1991). An additional problem with the IgG index is its basic premise that the selectivity of the protein transfer at the blood-CSF barrier is independent of the actual permeability condition. This concept has been shown to be incorrect and the IgG index, as well as the IgA and IgM indices, vary in a nonlinear fashion with progressive impairment of the barrier (Reiber and Felgenhauer, 1987). Therefore, Reiber and Felgenhauer (1987) developed a formula to calculate the intrathecally synthesized fractions of IgG, IgM, and IgA in the CSF. As with IgG, CSF IgM and IgA may be of serum origin or intrathecally produced. Indices for IgM and IgA can be calculated in the same fashion as for IgG (Fryden et al., 1978). However, because of high variability in normal IgM and IgA levels and the biological variation of these large molecules, the application of the same formula for IgM and IgA indices as used for the IgG index may only provide rough estimates (Reiber and Felgenhauer, 1987; Tipold et al., 1994). Qualitative assays of CSF immunoglobulins include agarose-gel electrophoresis, acrylamide immunoelectrophoresis, isoelectric focusing, and immunofixation. These tests separate the proteins into “bands” and provide information regarding the CSF protein composition. Although abnormal band patterns are not specific for a particular disease, they do indicate pathology and may indicate a type of disease. Abnormal band patterns may be detected even in patients with a normal IgG index. Thus, both quantitative and qualitative immunoglobulin assays are useful in the assessment of central nervous system disorders in both people and animals, particularly immunological or inflammatory diseases (Bichsel et al., 1984b; Deisenhammer et al., 2006; Fishman, 1992; Kjeldsberg and Knight, 1993). A variety of CSF antibody and antigen tests are available for viruses, fungi, rickettsia, protozoa, parasites, and other organisms (Berthelin et al., 1994a; Duarte et al., 2006; Dubey, 1990b; Jacobs and Medleau, 1998; Lunn et al., 2003; Madhusudana et al., 2004; Porter et al., 2004; Rossano et al., 2003). For antibody titers, two samples taken 2 weeks apart should be assayed. Because of inter-run variability, the samples should be assayed at the same time in the same analytical run. Interpretation of CSF antibody titers must take into account the possibility of passage of serum antibodies through a defective blood-brain/CSF barrier. Serum antibodies could be present because of disease, previous exposure to antigen, or vaccination. Ideally, the CSF/serum albumin index and IgG index are also determined (see Sections V.D.3 and V.D.4) to identify blood-CSF barrier dysfunction and intrathecal production of immunoglobulin. Intrathecal production of antigen-specific antibody (specific Ig) can be determined with an antibody index in the same fashion as intrathecal IgG production is detected with the IgG index. The formula is (Reiber and Lange, 1991): A modification of this formula accounting for large local synthesis of polyclonal IgG in the central nervous system may be necessary (Reiber and Lange, 1991). An antibody index > 1 suggests intrathecal production of the specific antibody (Munana et al., 1995; Reiber and Lange, 1991). Antibody indices have been calculated in human patients with a variety of diseases (Reiber and Lange, 1991). The diagnostic reliability of these indices and application to clinical veterinary medicine need further study. Antigen detection tests include immunoelectrophoretic techniques, agglutination tests, and enzyme-linked immunosorbent assay (ELISA) for bacterial antigens and latex agglutination for cryptococcal antigens. The polymerase chain reaction (PCR) procedures detect the presence of specific antigen DNA (or RNA) in CSF and can be highly sensitive, specific, and rapid (see Section V.C.5). The Gram stain, the Ziehl-Neelson acid-fast stain, and both aerobic and anaerobic cultures of CSF are time-honored methods for diagnosis of bacterial central nervous system infections. Bacteriological tests must be performed as soon as possible after CSF acquisition because some bacteria undergo rapid autolysis in the test tube. Additional tests such as the acridine orange stain for bacteria, tests for microbial antigens by counterimmunoelectrophoresis or agglutination techniques, and the G test for the broad spectrum detection of fungi (tests for (1,3)-β-D-glucan in most fungal cell walls) (Stevens, 2002) may also be useful (Fishman, 1992). PCR may be used to detect microbes in CSF (Finno et al., 2006; Peters et al., 1995; Stevens, 2002). Erythrocytes may be present in CSF samples because of subarachnoid hemorrhage or, more commonly, because of traumatic puncture. Blood contamination resulting from traumatic puncture is a common problem during CSF collection and, depending on its degree, can interfere with cytological interpretation. Blood contamination is more likely to occur with lumbar puncture as opposed to cerebellomedullary cisternal puncture (Bailey and Higgins, 1985; Oliver and Lorenz, 1993; Thomson et al., 1990). Blood contamination is a source of leukocytes and hence can affect both the leukocyte count and the differential. In one study of CSF analysis in cats (Rand et al., 1990b), the total leukocyte count, the neutrophil percentage and the eosinophil percentage were positively correlated with the CSF erythrocyte count once this count exceeded 500 erythrocytes per microliter. However, there was no significant increase in total white blood cell count or alteration in the differential percentages with up to 500 RBC/μl of CSF. Numerous correction factors have been used to correct leukocyte counts for the effect of blood contamination and include the following: in people, 1 white blood cell per 700 red blood cells is subtracted from the total white blood cell count (Fishman, 1992); in dogs, 1 white blood cell per 500 red blood cells is subtracted from the total count (Bailey and Higgins, 1985); in cats, a maximum of one white blood cell per 100 red blood cells is subtracted (Rand et al., 1990b). A more accurate formula takes into account the actual white blood cell and red blood cells counts of the patient and hence compensates for any significant alterations in these counts (Fishman, 1992): where W is the white blood cell count of the fluid before blood was added (i.e., the corrected count), WBCF is the total white blood cell count in the bloody fluid, WBCB is the white blood cell count in the peripheral blood per microliter, and RBCF and RBCB are the numbers of red blood cells per microliter in the CSF and blood, respectively. Despite all of these elaborate corrections, our own experience is that many thousands of red blood cells in contaminated samples of CSF will frequently be observed without any accompanying white blood cells, suggesting that these correction factors may not be valid. This empirical observation has been made by others (de Lahunta, 1983). This lack of validity has been proven by several studies (Novak, 1984; Wilson and Stevens, 1977). In one article, blood contamination appeared to have little effect on white blood cell numbers, and the above correction formula was considered unreliable. The authors evaluated 91 samples from both normal and diseased animals where there were numerous red blood cells but no white blood cells. Some of the red blood cell counts exceeded 15,000 RBC/μL, but white blood cells were still absent (Wilson and Stevens, 1977). In another article, the authors concluded that the standard computations frequently overcorrect white blood cell counts in blood contaminated CSF, and the magnitude of the overcorrection may obscure disease in some instances—in eight infants with marked blood contamination but proven bacterial meningitis, correction computations normalized or overcorrected the white blood cell counts (Novak, 1984). The mechanism of this overcorrection was not defined, but it is clear that the presence of low numbers of neutrophils should not be immediately discounted when red cells are concurrently found (Christopher et al., 1988). A study of feline CSF (Rand et al., 1990a) also found that values for CSF total protein, lactate dehydrogenase, creatine kinase, IgG ratio, and γ-globulin percentage were affected by blood contamination. The CSF total protein value of blood-contaminated CSF may be corrected using the formula for white blood cell correction given previously but substituting the total protein levels of the bloody CSF and the serum for the corresponding white blood cell counts (Kjeldsberg and Knight, 1993). In people, bloody contamination of CSF with as little as 0.2% serum (equivalent to about 5000 to 10,000 RBC/ml) elevates the IgG index (Fishman, 1992). Normal CSF is clear and colorless, and has the consistency of water. In pathological conditions the clarity, color, or consistency may change. Cloudy or turbid CSF is usually due to pleocytosis; about 200 WBC/μl or 400 RBC/μl will produce a visible change. With these low levels of cellularity, the CSF may appear opalescent or slightly hazy. Microorganisms, epidural fat, or myelographic contrast agent may also produce hazy or turbid CSF. Although the term xanthochromia means yellow color, it has often been used to describe pink CSF as well. The color of CSF is most usefully described as (1) pink or orange, (2) yellow, or (3) brown. These colors correspond to the major pigments derived from red cells: oxyhemoglobin, bilirubin, and methemoglobin. Oxyhemoglobin is red in color, but after dilution in the CSF it appears pink or orange. Oxyhemoglobin is released from lysed red cells and may be detected in the CSF supernatant about 2 h after red cells enter the CSF. The level of oxyhemoglobin reaches its peak about 36 h later and disappears over the next 4 to 10 days. Bilirubin is yellow in color. Bilirubin is derived from hemoglobin and is formed by macrophages and other leptomeningeal cells that degrade the hemoglobin from lysed red blood cells. Bilirubin is detected about 10 h after red cells enter the CSF, reaches a maximum at about 48 h, and may persist for 2 to 4 weeks. Bilirubin is also the major pigment responsible for the abnormal color of CSF with a high protein content. Methemoglobin in CSF is dark yellow-brown. Methemoglobin is a reduction product of hemoglobin characteristically found in encapsulated subdural hematomas and in old, loculated intracerebral hemorrhages (Fishman, 1992; Kjeldsberg and Knight, 1993). Occasionally the CSF may be black tinged CSF in animals with melanin-producing tumors in the nervous system. Causes of a CSF color change other than red cell contamination include icterus resulting from liver disease or hemolytic disease, markedly increased CSF total protein level, and drug effects. Both free and conjugated bilirubin may be present in the CSF, although the amount of bilirubin in the CSF does not correlate well with the degree of hyperbilirubinemia. If the CSF protein level is increased, the color change will be greater because of increased amounts of the albumin-bound bilirubin. High CSF protein content alone can impart a yellow color to the CSF (Fishman, 1992; Kjeldsberg and Knight, 1993). The drug rifampin imparts an orange-red color to body fluids. Rifampin is 90% bound to protein; hypoproteinemia may result in rifampin staining of CSF in patients receiving this drug (Fishman, 1992).
I. INTRODUCTION
II. FUNCTIONS OF CEREBROSPINAL FLUID
III. CSF FORMATION, CIRCULATION, AND ABSORPTION
A. Anatomy of Brain-Fluid Interfaces
1. Blood-Brain Barrier
2. Blood-CSF Barrier
3. CSF-Brain Interface
B. CSF Formation
1. Choroidal and Extrachoroidal Formation
Species
Rate (μl/min)
Mouse
0.325
Rat
2.1–5.4
Guinea
pig 3.5
Rabbit
10
Cat
20–22
Dog
47–66
Sheep
118
Goat
164
Calfb
290
Monkey
28.6–41
Human being
350–370
2. Rate of CSF Formation
C. CSF Circulation
D. CSF Absorption
IV. CELLULAR COMPOSITION OF NORMAL CSF
A. Total Erythrocyte and Nucleated Cell Count
B. Differential Cell Count
1. Leukocytes
2. Other Cells
V. BIOCHEMICAL CONSTITUENTS OF NORMAL CSF
A. Ontogeny of CSF
B. Proteins in the Cerebrospinal Fluid
1. Albumin
2. Alpha and Beta Globulins
3. Gamma Globulins
a. IgG
b. IgM and IgA
4. Other Proteins
C. Glucose
D. Enzymes
E. Neurotransmitters
F. Other CSF Constituents
G. Concentration Gradient along the Neuraxis
VI. CSF COLLECTION AND ANALYTICAL TECHNIQUES
A. Collection
1. General techniques
2. Collection site
3. CSF collection from the Cerebellomedullary Cistern
B. Physical Examination: Clarity, Color, and Viscosity
C. Cytological Analysis
1. General Techniques
2. Total Leukocyte and Erythrocyte Counts
3. Cytological Examination
4. Immunocytochemistry
5. Polymerase chain reaction
D. Protein Analysis
1. Measurement of CSF Total Protein
2. CSF Protein Fractionation
3. Albumin and the CSF/Serum Albumin Index
4. Quantitative Measurement of Immunoglobulins
a. IgG and the IgG/Albumin Index
b. IgM and IgA Indices
5. Qualitative Immunoglobulin Assays
E. Antibody/Antigen Tests
F. Microbial Tests
G. Blood Contamination
VII. GENERAL CHARACTERISTICS OF CSF IN DISEASE
A. Physical Characteristics: Clarity, Color, and Viscosity
1. Clarity
2. Color
3. Viscosity
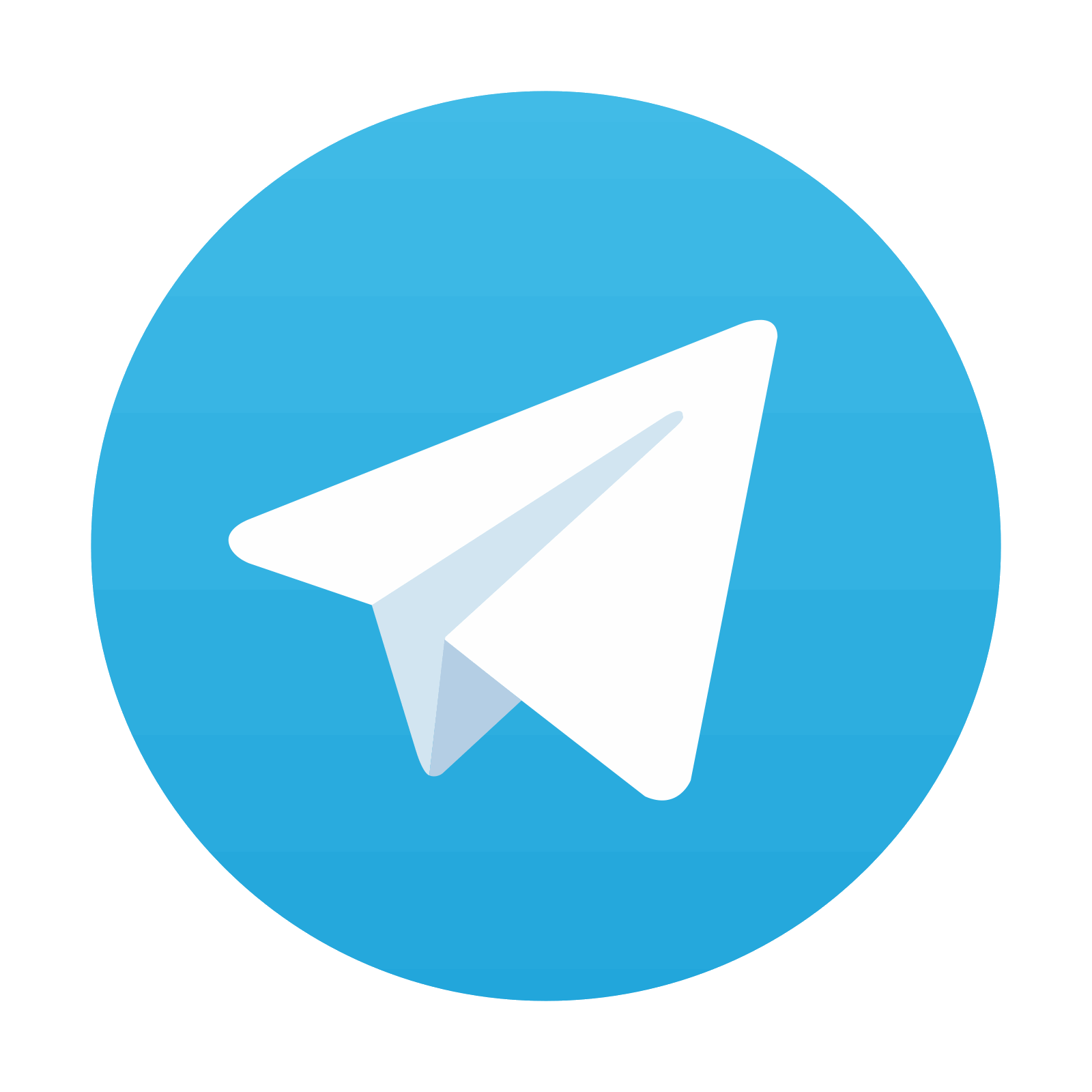
Stay updated, free articles. Join our Telegram channel
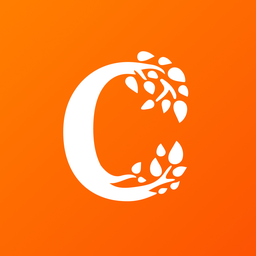
Full access? Get Clinical Tree
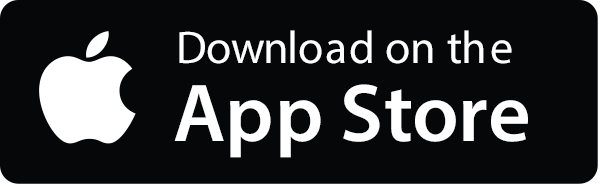
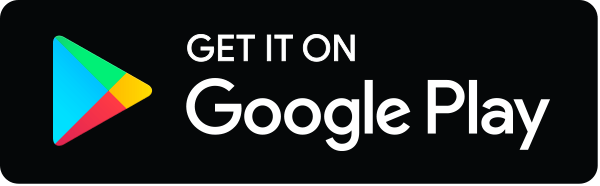