CHAPTER 1 The pathogenesis of this disease might be stated in an abbreviated form like the following: Note that various levels of diagnosis were made in the previous scenario. Diagnosis is a concise statement or conclusion concerning the nature, cause, or name of a disease. The accuracy of a diagnosis is limited by the evidence (lesions) available for study. A clinical diagnosis is based on the data obtained from the case history, clinical signs, and physical examination. It often suggests only the system involved, or it provides a list of differential diagnoses. The differential diagnosis (often termed rule outs in clinical medicine) is a list of diseases that could account for the evidence or lesions of the case. A clinical pathologic diagnosis is based on changes observed in the chemistry of fluids and the hematology, structure, and function of cells collected from the living patient. A morphologic diagnosis or lesion diagnosis is based on the predominant lesion(s) in the tissue(s) (see Chapter 3 and Fig. 3-23). It may be macroscopic (gross) or microscopic (histologic) and describes the severity, duration, distribution, location (organ or tissue), and nature (degenerative, inflammatory, neoplastic) of the lesion. An etiologic diagnosis is even more definitive and names the specific cause of the disease. A disease diagnosis is equally specific and states the common name of the disease. Components of Normal Cells and their Vulnerabilities The early pathologists Morgagni and Bichat emphasized the importance of organs and tissues as the seat of disease. Virchow later focused on individual cells as the primary cause of abnormal function and structure associated with diseases. Before we can interpret lesions of sick cells, it is essential that we understand normal cell structure and function. The cell can be visualized simplistically as a membrane-enclosed compartment, subdivided into numerous smaller compartments (organelles) by membranes (Fig. 1-1). This vast interconnecting system of membrane-bound spaces is termed the cytocavitary network. The function of these organelles is largely determined by the type and quantity of specific enzymes associated with each membrane and in the cytoplasmic matrix. Cell membranes are a fluid phospholipid bilayer penetrated by numerous specific proteins (Fig. 1-2). The two main biologic functions of these membranes are (l) to serve as selective barriers and (2) to form a structural base for the enzymes and receptors that determine cell function. Cell membranes form the boundaries of many organelles and separate them from the cytosol. Fig. 1-2 Fluid mosaic model of cell membrane structure. The plasma membrane is the cell’s first contact with injurious agents. Microvilli and cilia are specialized areas of the plasma membrane and are often specifically altered in disease (see Fig. 1-1). Plasma membranes separate the interior of the cell from external surfaces, neighboring cells, or surrounding matrix. Surface proteins, such as fibronectin, play a role in cell-to-cell and cell-to-ECM interactions. Transmembrane proteins embedded in the phospholipid bilayer serve in a variety of structural, transport, and enzymatic functions essential to cell viability (Fig. 1-3). It is these transmembrane proteins that are often used by infectious microbes to enter or use cell systems during their life cycles, thus initiating a process that often results in injury to the host cell. Mitochondria (singular = mitochondrion) are the “powerhouses” of highly specialized eukaryotic cells. They are the site of fatty acid oxidation, the citric acid cycle, and oxidative phosphorylation. Transfer of electrons from reduced cytochrome oxidase to molecular oxygen is the final and critical step culminating in these catabolic pathways. Important structural components of a mitochondrion are the outer membrane, outer compartment, inner membrane, inner compartment (matrix), cristae, and mitochondrial DNA. Damage to mitochondria results in diminished adenosine triphosphate (ATP) production and if damage is unchecked, cell death (see Fig. 1-6). The nucleus is that portion of the cell responsible for storage and transmission of genetic information (see Fig. 1-1). Chains of DNA, complexed to protein, are chromatin. Areas of uncoiled chromatin (euchromatin) are active in the generation of messenger RNA (mRNA) for protein synthesis. Highly coiled chromatin (heterochromatin) is inactive in transcription. The outer nuclear membrane is continuous with that of the rough endoplasmic reticulum (RER). The nucleolus is a basic organelle of the nucleus and is composed of RNA, nucleolus-associated chromatin, and protein (see Fig. 1-1). It functions in the synthesis of ribosomal RNA (rRNA), essential in protein synthesis. The nucleolus can be basophilic or eosinophilic, and its prominence is a subjective measure of the cell’s synthetic activity. The RER is a network of intracellular membranes studded with ribosomes (Fig. 1-4). RER is prominent in cells producing large amounts of extracellular protein (e.g., reactive fibroblasts, hepatocytes, plasma cells, and pancreatic acinar cells). The RER is responsible for the basophilia of the cytoplasm because of the numerous ribosomes, which contain acid (i.e., RNA). Fig. 1-4 Membrane systems. Smooth endoplasmic reticulum (SER) is a tubular or vesicular form of cell membrane that lacks ribosomes (see Fig. 1-1). SER is the locus of enzymes that metabolize steroids, drugs, lipids, and glycogen. It gives the cytoplasm a pale, finely vacuolated appearance as viewed in the light microscope. The Golgi complex consists of several lamellar stacks or flattened sacs of membranes, vesicles, and vacuoles (see Fig. 1-4). It functions in the synthesis of complex proteins by the addition of carbohydrate molecules and in the production of secretory vesicles and lysosomes. Lysosomes are small membrane-bound vesicles laden with hydrolytic enzymes essential for intracellular digestion (see Fig. 1-1). They are discussed more completely as components of phagocytic cells. Peroxisomes are similar to lysosomes but also play a role in energy metabolism. These structures are composed of protein subunits and function in the cytoskeleton and in cell movement (Fig. 1-5). They have a prominent role in the mitotic spindle, cilia, microvilli, neurons, myocytes, and phagocytic cells. Many cell types besides muscles, for example, contain actin microfilaments. Fig. 1-5 Cytoskeleton. Although not part of the cell itself, the ECM and its integrity influences cell health and function (see Chapter 3 and Web Figs. 3-23 and 3-24). ECM includes basement membranes and interstitial matrices composed of various collagens, proteoglycans, and adhesive glycoproteins among a variety of other molecules that interact with cells by means of various integrin molecules. Basement membrane integrity, for example, is essential for the proper structure and functioning of epithelial cells. Other components of the ECM influence how cells grow and differentiate. Causes of cell injury are numerous and can be classified in a variety of ways. Some causes, such as physical trauma, viruses, and toxins, are clearly extrinsic, whereas others, such as spontaneous genetic mutations, are clearly intrinsic. Others, such as workload imbalance, nutritional abnormalities, and immunologic dysfunctions, can have components of both extrinsic and intrinsic mechanisms. General mechanisms of injury include ATP depletion (often caused by hypoxia), membrane damage (a result of a myriad of causes, including oxygen-derived free radicals), disturbances of cellular metabolism, and genetic damage (Fig. 1-6). Fig. 1-6 Cellular and biochemical sites of damage in cell injury. Cells respond to stimuli and stressors in a variety of ways to maintain homeostasis. Cell injury takes place when a cell can no longer maintain a steady state. Some types of cell injury, such as cell swelling, can be reversible if the extent and duration of injury are not excessive. But if the injury exceeds certain limits, cell death and irreversible change occur. Not all cell injury results in cell death. Cell injury may be sublethal and result in a variety of types of cell degenerations or accumulations and/or adaptations by the cell to the injury. In essence, cells or tissues respond to injury (or stress) in three important ways: (l) adaptation, (2) degeneration or intracellular or extracellular accumulations, and (3) death (Fig. 1-7). Pathologically, reversible cell injury is injury from which the cell can adapt or recover and thus return to normal or nearly normal function. Irreversible cell injury results in a dead cell. This distinction seems clear-cut, but the point at which a cell transitions from reversible cell injury to irreversible cell injury (i.e., “the point of no return”) has been a major research challenge for the past few decades and remains so today (Fig. 1-8). The lesions of reversible and irreversible cell injury are discussed in greater detail in subsequent sections; however, in summary, the cytomorphologic changes characteristic of irreversible cell injury include the following: Fig. 1-8 Postulated sequence of events in reversible and irreversible ischemic cell injury. • Mitochondrial swelling and vacuolization • Amorphous densities (likely calcium) in the mitochondria Hypoxia is one of the most common and important causes of cell injury and death (see Fig. 1-8). Hypoxia is a partial reduction in the O2 concentration supplied to cells or tissue; a complete reduction is referred to as anoxia. Oxygen is critically important for oxidative phosphorylation, especially in highly specialized cells such as neurons, hepatocytes, cardiac myocytes, and renal tubule cells. Hypoxia can result from inadequate oxygenation of blood as a result of heart failure or respiratory failure, loss or reduction of blood supply (ischemia), reduced transport of O2 in blood (e.g., anemia or carbon monoxide toxicity), and blockage of cell respiratory enzymes (cyanide toxicosis). The immune system may fail to respond to infectious agents and other antigens as a result of congenital or acquired defects of lymphoid tissue or their products (see Chapter 5). Examples of congenital defects are thymic aplasia of nude mice and combined immunodeficiency of Arabian foals. Affected animals may die at an early age from infection by opportunistic microorganisms. Acquired immunodeficiency disease may be transient and results from damage to lymphoid tissue by viral infection, chemicals, and drugs. Cell swelling, also called hydropic degeneration and by other names in different organ systems (e.g., cytotoxic edema in the central nervous system and ballooning degeneration in the epidermis), is the most common and fundamental expression of cell injury (Fig. 1-9). It is manifested as increased cell size and volume resulting from an overload of water caused by a failure of the cell to maintain normal homeostasis and regulate the ingress and excretion of water. It is accompanied by modification and degeneration of organelles. Mechanisms responsible for acute cell swelling usually involve damage to cellular membranes, failure of cellular energy production, or injury to enzymes regulating ion channels of membranes. Cell swelling occurs in response to loss of the cell’s homeostasis secondary to mechanical, hypoxic, toxic, free radical, viral, bacterial, and immune-mediated injuries. Fig. 1-9 The process of acute cell swelling (hydropic degeneration). The hepatotoxicities of CCl4 and chloroform (CHCl3) provide classic examples of cell membrane injury (Fig. 1-10). Toxic effects of CCl4 occur when the chemical is converted to the trichloromethyl radical, CCl3•, by the mixed-function oxidase system of the SER in hepatocytes. The toxic metabolite, CCl3•, next causes progressive lipid peroxidation of unsaturated fatty acids of cellular membranes, progressing from the SER to mitochondria and other cell membranes. Chloroform is toxic to hepatocytes when it is metabolized to the electrophilic metabolite, phosgene (COCl2•). The hepatic lesions associated with these two toxins are indistinguishable, and both may result in fatty liver. Fig. 1-10 Sequence of events leading to fatty change and cell necrosis in carbon tetrachloride (CCl4) toxicity. Besides toxins, other processes may cause cell membrane injury leading to acute cell swelling. The membrane-attack complex of serum complement (see Chapter 3) and the hemolysin of streptococci (streptolysin-O) penetrate cell membranes to form a channel for free passage of water, proteins, and electrolytes between intracellular and extracellular compartments. Affected cells are quickly lysed by water overload (hypotonic lysis). Cytotoxic effects of natural killer (NK) cells are mediated in part by the implantation of similar hollow protein-complexes into target cell membranes. The sequence of events in acute cell swelling caused by hypoxia or ischemia (see Fig. 1-8) is as follows: 2. Decrease of oxidative phosphorylation and ATP 3. Increased glycolysis, increased intracellular lactate, and depletion of glycogen stores 4. Failure of Na+-K+ pump as the result of ATP deficiency 5. Net influx of Na+, Ca2+, and H2O with loss of intracellular K+ and Mg2+ 6. Swelling of mitochondria and the cytocavitary network (RER, SER, Golgi, and outer nuclear membrane) 7. Detachment of ribosomes, clumping of nuclear chromatin, loss of microvilli, vesiculation of endoplasmic reticulum (ER), formation of membrane whorls (“myelin figures”) 8. Severe disruption of cell membranes, influx of Ca2+ into mitochondria and cytosol, overall cell enlargement, and clearing of the cytosol Gross Appearance: Acute cell swelling is recognized as pallor, organ swelling, and decreased specific gravity. For example, the liver will be pale and somewhat turgid (Fig. 1-11, A). The parenchyma of organs with capsules may bulge when incised. Fig. 1-11 Acute cell swelling, liver, mouse. Microscopic Appearance: The influx of water dilutes the cytoplasmic matrix and dilates organelles to give cells a pale, finely vacuolated appearance (cloudy swelling). Renal tubule epithelial cells bulge and impinge on the tubular lumen. Swollen hepatocytes and endothelial cells intrude upon and diminish vascular lumens. Although mechanisms of cell swelling are limited, variations in appearance may occur because of differences in cell type and cause of injury. Hydropic degeneration is a common term used for the microscopic appearance of acute cell swelling (Fig. 1-11, B). It occurs in endothelium, epithelium, alveolar pneumocytes, hepatocytes, renal tubular epithelial cells, and neurons and glial cells of the brain. Cytoplasm of affected cells contains translucent vacuoles that fail to stain for fat or glycogen (two other causes of vacuolar degeneration). These vacuoles represent swollen mitochondria and dilated cisternae of the Golgi and ER. Ballooning degeneration is an extreme variant of hydropic degeneration in which cells are greatly enlarged and the cytoplasm is basically a clear space (Fig. 1-12). Ballooning degeneration is typically seen in epidermal cells infected by epitheliotropic viruses (e.g., poxvirus). This lesion frequently progresses to the formation of vesicles or bullae (blisters) from lysis of the epidermal cells. These viral infections cause both degradation of cytoplasmic proteins (cytoplasmic proteolysis) and net flux of water into the cytoplasm. Fig. 1-12 Ballooning degeneration, papular stomatitis, oral mucosa, cow. Ultrastructural Appearance: As visualized with the electron microscope, swollen epithelial cells have lost and distorted cilia, microvilli, and attachment sites, as well as “blebbing” of cytoplasm at the cell surfaces. The cytoplasm is rarefied, and the cisternae of the ER, Golgi, and mitochondria are dilated. The cytocavitary network becomes fragmented into numerous vesicles. Proteins and Ca2+ precipitate in the cytoplasm and in organelles. Acute cell swelling in the central nervous system has other distinctive features (see section on Cerebral Edema in Chapter 14). Injured cells that can no longer regulate water and electrolytes are no better equipped to maintain other cell functions. Significance to the patient depends on the number of cells affected and the immediate importance of the lost cell function. Cells highly vulnerable to hypoxia and cell swelling include cardiac myocytes, proximal renal tubule epithelium, hepatocytes, and endothelium. In the central nervous system (CNS), besides endothelium, neurons, oligodendrocytes, and astrocytes also are swollen, and the process in the CNS is called cytotoxic edema (see Chapter 14). Swollen neurons fail to conduct nervous impulses, resulting in stupor or coma. Swollen myocardial cells contract with less force or with an abnormal rhythm. Swollen renal epithelium may not only fail to absorb and secrete but also may compress delicate interstitial blood vessels, resulting in further injury. Capillaries lined by swollen endothelium are prone to obstruction, exacerbating the lesions by worsening cellular hypoxia. Injured cells with abnormal membrane permeability may be detected by finding their specific cytoplasmic enzymes in serum. As we have just seen, major mechanisms of acute cell swelling are hypoxia, including ischemia, and membrane injury, often by toxins. Cell swelling can be reversible if the extent and duration of injury is not excessive. But if the injury exceeds certain limits (discussed shortly), cell death occurs (Fig. 1-13). Not all cell injury results in cell death. Cell injury may be sublethal and result in a variety of types of cell degenerations and/or adaptations by the cell to the injury. In essence, cells or tissues respond to injury (or stress) in three important ways: (l) adaptation (with or without accumulations or degenerative changes), (2) reversible injury (again with or without subcellular changes), and (3) death. In this section, we deal with cell death. Various types of cell adaptations, degenerations, and accumulations are addressed in subsequent sections. Fig. 1-13 Normal cell and the changes in reversible and irreversible cell injury. How then are we to use the term necrosis? Attempts are being made by toxicologic pathologists to use the term necrosis for the histologic changes that occur after cell death by either mechanism, using the terms oncotic cell death or apoptotic cell death when a distinction needs to be made. We attempt to adhere to this distinction here, but long-used terminology does not easily change. The next sections first discuss cell death after irreversible cell injury by hypoxia and cell membrane damage (oncotic necrosis), and then apoptosis or apoptotic necrosis (Fig. 1-14). Fig. 1-14 The sequential ultrastructural changes seen in necrosis (left) and apoptosis (right). Prevention of Ca2+ influx can reduce irreversible injury. The reactivity of free Ca2+ ion and its role as an intracellular messenger and enzyme activator are known, and these actions are thought to contribute much to the final demise of the cell in necrosis. What does Ca2+ do to cause the ultimate demise of many severely injured cells as it influxes from the extracellular space (Fig. 1-15)? At least one endogenous, membrane-bound phospholipase (phospholipase A) is activated by free Ca2+. Activated phospholipases then break down the normal phospholipids of the inner mitochondrial membrane and other cell membranes. These events then preclude any possibility for cell survival. Activation of phospholipases also generates arachidonic acid, the substrate for many lipid mediators of inflammation (to be discussed later). Therefore it is usual to see some degree of inflammation around foci of necrosis. In addition to phospholipases, Ca2+ also activates proteases that result in cytoskeleton and membrane damage, adenosine triphosphatases (ATPases) that accelerate depletion of ATP, and endonucleases that result in chromatin degradation. Irreversible injury to mitochondrial membranes appears to be the deathblow to the cell. As if this were not enough, cells injured by ischemia can also die by apoptosis because of the leakage of proapoptotic molecules from injured mitochondria.
Cellular Adaptations, Injury, and Death
Morphologic, Biochemical, and Genetic Bases
Basic Terminology
Types of Diagnosis
The Normal Cell
Cell Membranes
The lipid bilayer provides the basic structure and serves as a relatively impermeable barrier to most water-soluble molecules. (From McCance K, Huether S: Pathophysiology: the biologic basis for disease in adults and children, ed 4, St Louis, 2002, Mosby.)
Mitochondria
Nucleus
Nucleolus
Rough Endoplasmic Reticulum
The rough endoplasmic reticulum and Golgi apparatus are important organelles in cellular biosynthesis of proteins and glycoproteins inserted into cell membranes and used in and secreted from cells. Transcription, translation, assembly, modification, and packaging of these molecules occur in an orderly sequence from the nucleus to the cell membrane as shown. Alterations in one or more of these steps can result in cell injury and serve as the underlying pathogenesis of a disease process. (From Copstead L, Banasik J: Pathophysiology, ed 4, St Louis, 2010, Mosby.)
Smooth Endoplasmic Reticulum
Golgi Complex
Lysosomes
Microfilaments, Intermediate Filaments, and Microtubules
The complexity of and interrelations between intermediate filaments, microtubules, endoplasmic reticulum, and other cytoplasmic organelles that can be involved in the pathogenesis of diseases are shown. (From McCance KL, Huether SE: Pathophysiology: the biologic basis for disease in adults and children, ed 5, St Louis, 2006, Mosby.)
Extracellular Matrix
Causes of Cell Injury
ATP, Adenosine triphosphate; ROS, reactive oxygen species. (From Kumar V, Abbas A, Fausto N, et al: Robbins & Cotran pathologic basis of disease, ed 8, Philadelphia, 2009, Saunders.)
Note that although reduced oxidative phosphorylation and adenosine triphosphate (ATP) levels have a central role, ischemia can cause direct membrane damage. ER, Endoplasmic reticulum; CK, creatine kinase; LDH, lactate dehydrogenase; RNP, ribonucleoprotein. (From Kumar V, Abbas A, Fausto N: Robbins & Cotran pathologic basis of disease, ed 7, Philadelphia, 2005, Saunders.)
Oxygen Deficiency
Immunologic Dysfunction
Reversible Cell Injury
ATP, Adenosine triphosphate. (From Huether S, McCance K: Understanding pathophysiology, ed 3, St Louis, 2004, Mosby.)
Cell Membrane Injury in Acute Cell Swelling
RER, Rough endoplasmic reticulum; SER, smooth endoplasmic reticulum. (From Kumar V, Abbas A, Fausto N: Robbins & Cotran pathologic basis of disease, ed 7, Philadelphia, 2005, Saunders.)
Morphology of Acute Cell Swelling
A, Hepatic swelling in a mouse exposed to chloroform 24 hours previously. The accentuated lobular pattern and slight pallor in the liver on the left are the result of acute cell swelling (hydropic degeneration) and necrosis of centrilobular hepatocytes. The right liver is normal. B, Liver from a mouse with chloroform toxicosis. While many hepatocytes in the centrilobular areas (at right) are necrotic, several cells at the interface of normal and necrotic (arrows) are still undergoing acute cell swelling (hydropic degeneration). H&E stain. (Courtesy Dr. L.H. Arp.)
Cells infected by some types of virus, such as papular stomatitis virus, are unable to regulate their volume and swell at certain stages of the infection. These cells may become very large (ballooning degeneration) and eventually rupture. Some of the cells have viral inclusion bodies (arrows). H&E stain. (Courtesy Dr. M.D. McGavin, College of Veterinary Medicine, University of Tennessee.)
Significance and Fate of Acute Cell Swelling
Irreversible Cell Injury and Cell Death
Reversible injury is characterized by generalized swelling of the cell and its organelles, blebbing of the plasma membrane, detachment of ribosomes from the endoplasmic reticulum, and clumping of nuclear chromatin. Transition to irreversible injury is characterized by increasing swelling of the cell, swelling and disruption of lysosomes, presence of large amorphous densities in swollen mitochondria, disruption of cellular membranes, and profound nuclear changes. The latter include nuclear condensation (pyknosis), followed by fragmentation (karyorrhexis) and dissolution of the nucleus (karyolysis). Laminated structures (myelin figures) derived from damaged membranes of organelles and the plasma membrane first appear during the reversible stage and become more pronounced in irreversibly damaged cells. (From Kumar V, Abbas A, Fausto N: Robbins & Cotran pathologic basis of disease, ed 7, Philadelphia, 2005, Saunders.)
Cell Death
In apoptosis, the initial changes consist of nuclear chromatin condensation and fragmentation, followed by cytoplasmic budding and phagocytosis of the extruded apoptotic bodies. Signs of cytoplasmic blebs, accumulation of myelin figures representing damaged phospholipid membranes, and digestion and leakage of cellular components characterize necrosis. (From Kumar V, Abbas A, Fausto N, et al: Robbins & Cotran pathologic basis of disease, ed 8, Philadelphia, 2009, Saunders.)
Cell Death by Oncosis (Oncotic Necrosis)
Stay updated, free articles. Join our Telegram channel
Full access? Get Clinical Tree
Cellular Adaptations, Injury, and Death
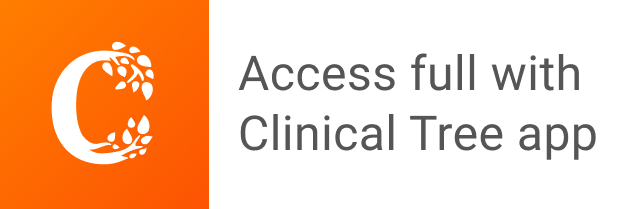