(1)
Center for Biomedical Genomics & Informatics, Department of Molecular & Microbiology, George Mason University, Manassas, VA, USA
Abstract
Cerebral autosomal dominant arteriopathy with subcortical infarcts and leukoencephalopathy (CADASIL) is a human genetic syndrome that causes multiple small strokes, due to a single-gene, autosomal dominant mutation with 100% penetrance. CADASIL mutations encode amino acid substitutions that increase or decrease the number of cysteines within the extracellular epidermal growth factor (EGF) repeat domain of the NOTCH3 receptor protein. Histological studies of CADASIL patients have shown a characteristic accumulation of granular osmiophilic material surrounding the arterial smooth muscle cells, and the degeneration and death of some of these smooth muscle cells. The adjacent endothelial cells appear normal. The result is occasional occlusion of small arteries, producing small infarcts throughout the body, particularly in subcortical white matter in the brain in persons over 50 years of age. Several experimental models related to CADASIL have been investigated, including transgenic mice, knockout mice, cell line expression systems, and Drosophila mutants. None of these models reproduce all the characteristics of human CADASIL, but taken together they have provided an increasingly detailed picture. It is clear that continuing NOTCH3 signaling is required in adult mammalian arterial smooth muscle cells to maintain their survival, differentiation, and normal responses to injury and mechanical stress, particularly in the smaller arteries. In these respects, the characteristics of CADASIL are consistent with a partial loss of NOTCH3 signaling. However, attempts to confirm a loss of NOTCH3 signaling by CADASIL alleles in cell culture have led to mixed results. CADASIL patients do consistently exhibit extracellular accumulation of granular osmiophilic material that contains the extracellular (but not the intracellular) domain of the NOTCH3 receptor. Moreover, the accumulation of the extracellular domain of NOTCH3 has been reproduced in some transgenic mice, as well as in a cell culture model. This gradual accumulation of extracellular aggregates of the NOTCH3 extracellular domain may interfere with NOTCH signaling and may help to explain the characteristically late onset of symptoms of CADASIL. In any case, the well-defined cellular and molecular defects in CADASIL provide a promising area for further research, and the location of the affected cell type could facilitate future drug treatments for this disease.
Key words
CADASILNOTCH3Stroke1 Introduction
Stroke is the third most common cause of death and the leading cause of long-term neurological disability in the world. Preventable (lifestyle-related) vascular risk factors for stroke (such as high blood pressure, atherosclerosis, obesity, and cigarette smoking) account for roughly 50% of the total risk for stroke (1). Genetic factors are believed to account for a significant proportion of the remaining stroke risk, in most cases through complex interactions of multiple genes, age, and environmental factors. Monogenic (single-gene) disorders account for approximately 1% of all ischemic strokes (1). Although these monogenic conditions are relatively rare, they provide valuable models for elucidating the biology of stroke and cerebrovascular disease, as well as the accompanying symptoms such as vascular dementia. The genes responsible for a number of inherited vascular diseases have been identified in recent years (2).
Cerebral autosomal dominant arteriopathy with subcortical infarcts and leukoencephalopathy (CADASIL) is the best known and probably the most common of the monogenic disorders that cause stroke and vascular dementia (3). CADASIL alleles are genetically dominant and have a penetrance of 100% (i.e., all individuals carrying one or more copies of the mutant allele will eventually exhibit this syndrome). Moreover, the molecular nature of the lesions within the NOTCH3 gene (4) are equally well defined – virtually all the mutations that cause CADASIL in humans result in an odd number of cysteine amino acids within the epidermal growth factor (EGF) repeats in the extracellular domain of the NOTCH3 receptor protein, possibly causing mispairing of disulfide bonds (5). An odd number of cysteines in the extracellular EGF repeats also cause a unique phenotype (not caused by other amino acid substitutions) in Drosophila Notch (6).
The high penetrance of the mutations that cause CADASIL, and their well-defined molecular basis, suggest that the study of this disorder has advanced to the point where animal models are appropriate (6). However, recent results from these animal models have been mixed at best. To put these results into perspective, it will be necessary for us to first briefly review what is known about the clinical characteristics of CADASIL, the molecular mechanisms of Notch signaling, and the role of this Notch signaling in the development and maintenance of blood vessels.
1.1 Clinical Characteristics of CADASIL: Pathology and Prognosis
CADASIL is a degenerative vascular disease that typically first causes noticeable symptoms in middle-aged individuals. Affected individuals exhibit a variety of symptoms, including migraines, mood disorders, recurrent subcortical ischemic strokes, progressive cognitive decline, dementia, and premature death. The histological characteristics of CADASIL include the progressive degeneration of smooth muscle cells (SMCs) in small arteries, the narrowing of small arteries, the accumulation of granular osmiophilic material (GOM) within the SMC basement membrane, and small, localized lesions throughout the body, particularly in the white matter of the brain, that are apparently due to multiple, small lacunar infarcts (3, 7). GOM accumulation in arterial SMC is one of the most distinguishing features of CADASIL, in small arteries throughout the body (7, 8). GOM accumulation in CADASIL patients does occur in larger arteries, although to a lesser extent (9). GOM accumulation in CADASIL patients has never been reported in veins. Another distinguishing feature of CADASIL is lacunar infarcts in the anterior temporal white matter and external capsule. Strokes involving the territory of a large artery have occasionally been reported in CADASIL patients, but are probably coincidental (10).
CADASIL is a relatively rare condition, but the number of published reports has been increasing in recent decades. The prevalence of CADASIL mutation carriers (including those who are asymptomatic at present) has been estimated to be 4 per 100,000 adults in Western Scotland (11). In most areas, CADASIL is underreported, due to the time, effort, expense, and multiple areas of expertise required to establish a definitive diagnosis. For example, a false-negative report of no family history of relevant symptoms is often incorrectly obtained on the initial patient interview (12). In addition, an initial observation of widespread lesions of white matter is often misdiagnosed as multiple sclerosis (13, 14). Clinical DNA sequencing, if undertaken at all, is often limited to exons 3 and 4 of the NOTCH3 gene, where the first mutations in CADASIL were found (5, 15). However, mutations that cause CADASIL have been found throughout the EGF repeat domain, that is in exons 2–24, corresponding to EGF repeats 1–34 of the NOTCH3 gene (8, 16–25). Another reason that CADASIL is underreported is that patients with CADASIL present with a wide variety of initial symptoms and diagnoses, including acute encephalopathy (26, 27), adjustment disorder (28), affective disorders (29), angiitis (30), bipolar disorder (31), cognitive impairment (32), coma (27), depression (32), dizziness and vertigo (33, 34), facial dystonia (35), impaired visual physiology (36, 37), intermittent neurological symptoms (38), migraine headaches with aura (28, 29, 32), multiple sclerosis (39), Parkinson’s disorder (40), postpartum psychiatric disturbance (41), presenile dementia (42), schizophrenia (43), seizures (32), subcortical strokes (32, 42), and sudden hearing loss (44).
The age at onset of the first CADASIL symptoms has varied from 26 to 70 years of age (28, 34). In most cases, the age at first diagnosed stroke is in the 40s (45) or 50s (46). Relatively few individuals with CADASIL experience their first stroke in the 20s or 30s (47). After the first observable stroke, affected individuals show a progressive decline in cognitive (48) and motor functions (28, 46), including periods of chronic progression, acute worsening, stabilization, and improvement (49). Most (78%) CADASIL patients eventually become completely unable to care for themselves, but they can live into their 60s or 70s if they are given sufficient care (45, 46). The life expectancy of CADASIL patients varies between families and nationalities, but is consistently below the average for their national region.
CADASIL is genetically dominant and has a penetrance of 100%; in other words, all individuals carrying the mutant gene eventually exhibit the disease and its progression. Nevertheless, there is variation in symptoms, and some of this variation is correlated within families. For example, the size and progression of CADASIL brain lesions are correlated within families, leading to an estimated heritability of 0.6–0.7 (50). Linear regression analysis confirmed that these variations in T2 lesion volume were age-dependent, but did not appear to be accounted for by the specific identity of the NOTCH3 mutant allele, suggesting that other genes modify the severity of the CADASIL phenotype (50). Consistent with this possibility, anecdotal reports suggest that vertigo may be experienced more often by CADASIL patients in Asia, while migraine may be experienced more often by CADASIL patients in Europe (34). A few particular CADASIL alleles may increase the likelihood of lesions of the corpus callosum (51), or increase the rate of disease progression (46). Nevertheless, large CADASIL families often include both affected individuals with frequent migraine headaches, as well as other CADASIL patients without migraine headaches. The psychiatric manifestations of CADASIL families also frequently vary within families, rather than between families (28).
CADASIL patients are generally heterozygotes, but a single exception to this rule is known – a 54-year-old homozygous CADASIL patient (47). Comparison to age-matched CADASIL patients (all carrying the same R133C allele as the homozygous patient) showed that the homozygous patient had the earliest age at first stroke (compared to nine heterozygotes), was the most severely affected on most neuropsychological tests (compared to eight heterozygotes), had the most severe findings by MRI (compared to seven heterozygotes), and had the greatest accumulation of granular osmiophilic material (GOM) (compared to one heterozygous patient). The homozygous patient also had two heterozygous sons whose age at first stroke was close to their father’s (47). Moreover, one of the heterozygous patients showed more rapid progression than the homozygous patient, and was so severely affected that he could not be included in the neuropsychological or MRI tests. In other words, the symptoms showed by the homozygous CADASIL patient were within the range seen in CADASIL heterozygotes, although near the severe end of the scale (47).
CADASIL patients have significantly reduced middle cerebral artery basal blood flow velocity, as well as significantly reduced cerebrovascular CO2 reactivity. Both these arterial defects become more severe with age and/or disease progression (52).
1.2 Genetic Nomenclature
As mentioned above, “CADASIL” is not a gene name; rather it is an acronym for the name of a genetic disease caused by certain mutations in the human NOTCH3 gene. This review includes similar mutations in human, rat, mouse, fruit fly, and cell culture studies, each of which has its own system of genetic nomenclature (53). Accordingly, specific references to human genes and proteins will be shown in all capital (Roman) letters, but gene names in other species will be italicized, and the corresponding names of specific proteins in those species will be shown in all capital (Roman) letters. General references to genetic pathways and signaling mechanisms will be shown in conventional text (neither italicized nor all capital letters).
2 Molecular Mechanisms of Notch Signaling
The Notch signaling pathway is a short-range channel of communication between adjacent cells that is involved in many fundamental aspects of multicellular life, including proliferation, stem cells and stem cell niche maintenance, cell fate acquisition, differentiation, cell death, and even the consolidation of long-term memory (54–60). Notch signaling is highly conserved throughout the animal kingdom. Both the Notch receptor and its ligands (known as Jagged in mammals) are transmembrane proteins with large extracellular domains, consisting primarily of EGF-like repeats. In mammals, the Notch receptor is processed during protein maturation by protease cleavage (also known as S1 cleavage), and is normally located at the cell surface as a heterodimer (one subunit contains a transmembrane segment). In other words, the extracellular domain associates noncovalently with the membrane-tethered intracellular domain (the latter includes a small extracellular stub). The extracellular domain of the Notch receptor usually contains 36 EGF-repeats, as well as three cysteine-rich Notch/LIN-12 repeats (LNG domain). EGF repeats are a rather common sequence motif in proteins and often bind to calcium ions, which may be necessary for the proper folding of the adjacent EGF repeat (61).
The EGF repeats in the Notch extracellular domain are posttranslationally modified by an O-fucosyltransferase (OFUT1) that attaches fucose specifically to certain serine and threonine amino acids that are required for Notch signaling (62). These fucose side chains are then extended by the “Fringe” family of enzymes, which have a fucose-specific β1,3 N-acetylglucosaminyl transferase activity and initiate elongation of the O-linked fucose during Notch processing in the Golgi apparatus (63–65). Both OFUT1 and Fringe are capable of producing both positive and negative effects on Notch signaling (62, 64). Fringe binds to Notch through the EGF repeats 22–36, as well as the LIN-12 repeats (65). Extension of fucose side chains by Fringe modifications modulates the preference of the Notch receptor for its ligands Delta and Jagged/Serrate (66–69).
In mammals, there are four Notch receptor genes (Notch1, Notch2, Notch3, and Notch4), all of which encode single-pass transmembrane proteins. These interact with a few ligands, which are also single-pass transmembrane proteins: JAGGED1, JAGGED2, DELTA-LIKE1, DELTA-LIKE3, and DELTA-LIKE4. Notch receptor proteins are located at the cell surface as a heterodimer due to proteolytic cleavage at the S1 site, as mentioned previously. Ligand binding leads to a second proteolytic cleavage of the Notch receptor at the S2 site (on the extracellular surface of the transmembrane segment). S2 cleavage is followed by endocytosis of the Notch extracellular domain, still bound to its protein ligand (70). This endocytosis of the Notch receptor and its ligands is regulated at multiple levels and is essential for both receptor/ligand activation and subsequent degradation (71–74). The membrane-tethered intracellular domain then becomes susceptible to S3 cleavage within the transmembrane segment, releasing the intracellular domain. The freed intracellular domain binds to the CSL (CBF1/Su(H)/LAG1) family of transcription factors (called CBF1/RBP-Jκ in mammals), along with its coactivator Mastermind, translocates to the nucleus, and regulates the transcription of target genes (54, 74–77). In most (perhaps all) cases, all four Notch receptors appear to activate this same intracellular transcription factor complex.
2.1 The Role of Notch Signaling in the Development of Blood Vessels
Genetic studies in vertebrate model systems have revealed a signaling cascade that is responsible for determining arterial and venous cell fates in embryonic blood vessels. This cascade is initiated by vascular endothelial growth factor A (Vegfa) signaling (78), which in turn induces signaling through the Notch1 pathway (79, 80). The Notch and Vegf pathways also regulate blood vessel sprouting (81). Within the developing dorsal aorta, the loss of Notch signaling leads to loss of artery-specific molecular markers, as well as abnormal expression of venous markers (82). Conversely, the ectopic activation of Notch signaling leads to repression of venous cell fate (82). Notch1 is normally expressed in arteries but not in veins (83). Moreover, Notch3 and its ligands Jagged1 and Jagged2 are expressed in arterial SMCs (but not in veins), while Notch4 and Delta4 are expressed in arterial endothelial cells (but not in veins) (83–86). Adult vascular phenotypes can also be produced by gain-of-function Notch4 alleles (87). Notch2 was not detectable in the circulatory system. Only Notch4 and Delta4 were detectable in capillaries (83).
After the initial development of the blood vessels, the SMCs within those blood vessels remain able to undergo significant changes in phenotype in response to cell signaling events and changes in local environmental conditions (88). Several different Notch genes are involved in these cell signaling events, and these Notch genes appear to have somewhat different roles. For example, Notch1 (87, 89–91) and Notch4 (90, 92, 93) play essential roles in embryonic angiogenesis and vascular remodeling, while Notch3 functions primarily in the postnatal development and plasticity of SMCs. Taken together, these results indicate that Notch signaling in the circulatory system is required for the normal development and maintenance of arteries and capillaries, but not veins.
In normal mice, Notch3 is expressed postnatally, in arteries but not in veins, and in SMCs, but not endothelial cells (87, 94, 95). In mouse Notch3 – / – knockouts, the embryonic stages of vascular development appear to be normal, and these mice develop into viable and fertile adults (96, 97). Mouse Notch3 – / – knockouts do have defects in the postnatal maturation of arterial SMCs (87, 95), and possibly reduced numbers of thymocytes in the immune system (98). Several lines of evidence (morphological, developmental, gene expression studies) indicate that arterial SMCs in mouse Notch3 – / – knockouts adopt a venous SMC identity (87, 95). For example, adolescent and adult Notch3 – / – mutant mice exhibited pronounced defects in arterial maturation that were not evident at birth, including enlarged arteries with a relatively thin and disorganized arterial SMC layer. The abnormal SMC layer was not due to alterations in cell proliferation or cell death, as shown by assays of the percentage of presumptive arterial SMCs that were entering mitosis or were labeled for apoptotic markers. Rather, presumptive arterial SMCs appear to have adopted the identity of normal vein SMCs, based on their morphology as well as their failure to express artery-specific molecular markers. In contrast, the endothelial cells in Notch3 – / – arteries express normal levels of several artery-specific endothelial cell markers, indicating that the arterial identity of the endothelial cells remains normal in Notch3 – / – knockouts. In spite of the apparently complete transformation of the identity of their arterial SMCs, Notch3 – / – mice did not show any obvious brain pathology on histological examination by 12 months of age. Later ages were not examined (95).
Additional observations suggest that the effects of Notch3 differ between small arteries, vs. medium-sized or large arteries (95, 99). The SM22α gene is normally expressed in all SMCs, but arterial-specific regulatory elements have been identified within the SM22α promoter, which were used to construct an arterial-specific promoter driving the expression of a LacZ reporter gene (100). In the large and medium-sized arteries of normal mice, the expression of this SM22α–LacZ reporter gene reached a peak at postnatal day 6 (P6), and remained at a high level thereafter. In the small arteries of normal mice, expression of the SM22α–LacZ reporter gene was barely detectable at P6, but increased later to reach its peak value at P28, in adolescent mice (99, 101). In the large and medium-sized arteries of Notch3 – / – knockout mice, the expression of SM22α–LacZ was normal until P6, after which expression declined. In the small arteries of Notch3 – / – knockout mice, SM22α–LacZ was not expressed at any age (99). In other words, the maturation of arterial SMCs in larger arteries after P6, and in smaller arteries at all ages, required NOTCH3 signaling. Taken together, these results from mouse genetics indicate that NOTCH3 signaling is: (i) required in arteries but not in veins, (ii) required in SMCs but not in endothelial cells, (iii) required for postnatal maturation of SMCs but not for prenatal development of SMCs, and (iv) has a greater effect on small arteries than on larger arteries. These characteristics are remarkably similar to the symptoms of CADASIL, which suggests (but does not necessarily prove) that CADASIL may be caused by (a) defect(s) in NOTCH3 signaling.
2.2 The Role of Notch Signaling in the Response to Vascular Injury
The neointima is a thickened layer of vascular SMCs that forms after vascular injury, presumably by proliferation of SMCs. This formation of the neointima was significantly decreased in Hey2 – / – knockout mice, suggesting that Notch signaling was required for normal formation of the neointima (102). Consistent with this view, primary aortic SMCs from Hey2 –/– knockout mice were found to proliferate at a reduced rate in cell culture (102), and expression of activated forms of Notch1 or Notch3 enhanced the proliferation of adult rat vascular SMCs in culture (103). Activated forms of Notch1 or Notch3 also inhibited apoptosis and cell migration (103). The effects of activated Notch3 on SMC proliferation depended on the details of cell culture conditions (84).
The arterial expression of several genes in the Notch pathway, including Notch1 and Notch3, as well as Notch ligands (Jag1 and Jag2) and downstream transcription factors (Hey1 and Hey2) are downregulated within the first 2 days after experimental vascular injury, but are later upregulated 7–14 days after injury (84, 104–106). During the downregulation of NOTCH1 and NOTCH3 receptor expression by mechanical strain in vitro, SMC proliferation was reduced and apoptosis increased (107, 108). Conversely, genetically forcing overexpression of the NOTCH1 or NOTCH3 intracellular domain (during mechanical strain in vascular SMCs in vitro) increased proliferation and decreased apoptosis (107, 108). Taken together with the developmental studies (see above), these observations suggest that Notch signaling is required to maintain the normal ability of arterial SMCs to withstand the mechanical strains produced by high and variable arterial pressures.
3 The Molecular Details: CADASIL Mutations and Granular Osmiophilic Material
Almost all the mutations that cause CADASIL in humans result in the gain or loss of a cysteine residue in one of the 34 EGF-like repeats in the extracellular domain of the NOTCH3 receptor (5, 109–116). In a carefully controlled study, 125 patients whose CADASIL diagnosis was confirmed by ultrastructural analysis of skin biopsy specimens were subjected to sequencing of NOTCH3 exons 3–4, and then (if no mutation was found) to further sequencing of exons 2–24. The results showed that patients with a positive skin biopsy had mutations that caused an odd number of cysteines in the EGF repeat domain in 120/125 cases; in the other five cases, no mutation was found. Of the mutations in the EGF repeat domain, 69% were located in exons 3–4 and 86% were located in exons 2–6 (8). Two of the mutations in exon 4 were associated with significantly faster disease progression, but four other mutations in exon 4 were not (46).
Very rarely, patients with some of the characteristics of CADASIL have been found to have amino acid substitutions in the NOTCH3 gene that do not change the number of cysteines in the EGF repeat domain (113, 117, 118). However, these may be polymorphisms unrelated to the disease condition. There is no published case in which these patients have been subjected to a detailed pedigree analysis to show cosegregation of a disease condition with the amino acid substitution. Therefore, the pathology in these cases may be caused by other genes, or even nongenetic factors. It is known that mutations in other genes can cause small vessel angiopathies (119). Moreover, it is also clear that amino acid sequence polymorphisms in the NOTCH3 gene are present in the human population that do not contribute to cerebrovascular disease (120).
The strikingly nonrandom nature of the mutations that cause CADASIL, and particularly the absence in CADASIL patients of frameshift mutations or deletions of the NOTCH3 gene, strongly suggests that the mutations that cause CADASIL are not simply NOTCH3-null alleles (5, 6, 121). A similar argument has been advanced with respect to cysteine mutations in the EGF repeat domain of the Notch gene in Drosophila, where more powerful genetic tools are available (see next section).
In electron microscope studies, the small arteries of CADASIL patients show extracellular aggregates of GOM in the vicinity of arterial SMCs (122). GOM are typically rounded profiles about 0.1–0.5 µm in diameter (94, 123), are often located in proximity to the basement membrane, and are sometimes located adjacent to the extracellular surface of the SMC plasma membrane, or even in isolation (i.e., within the artery, but not close to any particular structure) (94). It has been shown by immunohistochemical methods that the extracellular domain of the NOTCH3 protein is present in substantial amounts in these GOM aggregates, but the intracellular domain of the NOTCH3 protein is not present (94, 123). In experiments based on conventional embedding methods, the NOTCH3 extracellular domain appeared to be confined to surfaces of the GOM adjacent to SMC plasma membranes (94). However, postembedding immunogold electron microscopy with cryofixed and freeze-substituted biopsies (which allows better antibody access to the interior of molecular aggregates) has shown convincingly that the NOTCH3 extracellular domain is actually uniformly distributed throughout the interior of the GOM (123).
When biopsies of CADASIL arteries were analyzed on SDS polyacrylamide gels under reducing conditions, the results showed selective (and abnormal) accumulation of the extracellular domain of NOTCH3 (210 kDa), which was present in high levels. The 280 kDa NOTCH3 precursor and the 97 kDa intracellular domain showed no evidence of accumulation in these CADASIL biopsies. Under nonreducing conditions, the accumulation of NOTCH3 extracellular domains in CADASIL biopsies migrate in higher molecular weight form(s), suggesting the formation of abnormal disulfide bridges (94). Moreover, similar high molecular weight forms were also observed in a cell culture model of CADASIL, in which amino acid substitutions causing the gain or loss of a cysteine in a peptide portion of the extracellular domain of NOTCH3 were shown to be both necessary and sufficient to form abnormal, cross-linked homodimers of the NOTCH3 extracellular domain, as well as heterodimers of the NOTCH3 extracellular domain with an enzyme named “Lunatic Fringe”(25). These results suggest that Lunatic Fringe may normally have an unpaired cysteine, or alternatively that the unpaired cysteine in CADASIL may disrupt disulfide bond formation in adjacent proteins (25, 94). In any case, all the cysteine mutations tested in cell culture showed small but consistent decreases in the elongation of fucose side chains by Fringe (25). These Fringe modifications are known to modulate the preference of the Notch receptor for its ligands Delta and Jagged/Serrate (66–69).
These results raise several possible (although not mutually exclusive) scenarios. One possible scenario is that aberrant dimerization of the NOTCH3 extracellular domain may interfere with its normal reuptake, leading to the gradual accumulation of extracellular aggregates (GOM) that are characteristic of CADASIL and may interfere with binding to potential Notch ligands (25, 94, 123). Another possible scenario is that cross-linking of the NOTCH3 extracellular domain to enzymes in the Fringe family may inhibit the latter enzymes, which in turn are known to modulate the binding specificity of Notch for its various ligands (74). A third possible scenario is suggested by the observation that Notch signaling and Lunatic Fringe gene expression are coupled together, in an oscillatory negative feedback loop that plays key roles in mammalian development (124). Feedback between NOTCH3 signaling and Lunatic Fringe enzyme activity could gradually magnify the abnormalities in older CADASIL patients, perhaps leading to age-dependent pathology that is unrelated to arteriosclerosis.
4 Drosophila Models: Cysteine Mutations in the Extracellular EGF Repeat Domain of Notch
Drosophila has a single Notch gene, with essentially the same structure and function(s) as mammalian Notch genes (74). Hundreds of Drosophila Notch mutations are known, many of which have been classified by genetic (fine-structure) map location and phenotype into categories such as Abruptex, Confluens, notchoid, facet, and split (125). The Abruptex (Ax) class of Notch alleles have a characteristic, tissue-specific dominant visible phenotype (shortened, incomplete wing veins), and have been further subdivided into three subcategories, of which the recessive lethal subcategory (such as N Ax59b , N Ax59d , and N AxM1 ) have cysteine substitutions in the extracellular EGF repeats and thus may provide a model for CADASIL (6). These “lethal Abruptex” alleles have been characterized genetically as “loss-of-function” alleles, but in addition they also show particular phenotypes (for example in the initiation of proneural cluster development) that are characteristic of dominant negative Notch alleles (126). These results are not contradictory, because antimorphs (dominant negative alleles) are expected to have loss-of-function phenotypes, as well as an abnormal gain-of-function that allows them to interfere with the wild-type allele (in heterozygotes) (127).
This was clearly demonstrated for one of the lethal Abruptex alleles (N Ax59b , which corresponds to the cysteine substitution C972G in the EGF repeat 24 of the Notch gene), and was characterized as a loss-of-function allele in some respects (126), but appeared to be an antimorph (dominant negative) in other assays (128). Heterozygotes with one copy of the cysteine substitution C972G have the typical dominant visible phenotype that defines Abruptex alleles (shortened, incomplete wing veins). The addition of a downstream frameshift produced a double mutant allele that encoded a truncated protein and completely lacked Notch function (128). Heterozygotes carrying one copy of the double mutant allele lost the characteristic dominant visible Abruptex phenotype (see above), but gained an alternative dominant visible phenotype that is seen in heterozygotes of null Notch alleles or deletions of the Notch gene (notches in the wing margins) (125, 128). Therefore, the dominant visible Abruptex phenotype is caused in this case by an abnormal function, probably an antimorph (dominant negative) (126, 128).
Another possible Drosophila model for CADASIL is provided by the notchoid-3 mutation (N nd3 , previously known as nd3 or fa no ), which has a cysteine substitution in EGF repeat 2 of the Drosophila Notch gene (76). In other words, this cysteine substitution is located within the amino terminal region (EGF repeats 1–6) of Drosophila Notch, corresponding to the region of human NOTCH where the majority of CADASIL mutations are found (5, 8). Thus, analysis of N nd3 may help to elucidate why human CADASIL mutations are clustered in this area (76). On the other hand, CADASIL mutations have been found essentially throughout the EGF repeat domain of the human NOTCH3 gene (8, 16–25), including the EGF repeats 24–29 where most Abruptex mutations are located (125). Moreover, notchoid alleles have a negligible dominant phenotype (125) and other notchoid alleles map to the intracellular domain of Notch (129); in other words, the overall notchoid subgroup of alleles cannot be comparable to CADASIL. The lethal Abruptex subgroup of alleles does appear to be comparable to CADASIL, at least in the sense that the alleles in this group correspond to cysteine substitutions in the extracellular EGF repeats, and all share a unique, tissue-specific, dominant visible phenotype. One difference is that the lethal Abruptex alleles do appear (so far) to map to a more limited domain (EGF repeats 24–29) than CADASIL alleles (EGF repeats 1–34). However, EGF repeats 22–36 are involved in binding to Fringe (65), which as noted above, accumulates as a heterodimer with NOTCH3 in cell culture models of CADASIL (25).
In any case, Wesley and colleagues found that NOTCHnd3 receptors in Drosophila tissue culture cells showed a cold-sensitive reduction in Notch signaling and showed a reduced rate of internalization (76). Interestingly, both NOTCHnd3 and NOTCHAx59d receptors also accumulated to abnormally high protein levels and showed apparently similar abnormal distributions (low fraction available for cell-surface biotinylation) (76). The detailed molecular consequences of cysteine substitutions on Notch protein processing may be somewhat different in Drosophila than in vertebrates, because in Drosophila, the colinear full-length form (not the heterodimeric form as in vertebrates) is the predominant form of Notch receptor (130). Nevertheless, the finding that Drosophila Notch EGF cysteine substitutions cause a reduction in Notch signaling, as well as increased Notch protein accumulation and reduced Notch protein internalization, are all rather similar to the characteristics of CADASIL, particularly given that the accumulation of NOTCH3 in GOM is now recognized as being sufficiently specific and reproducible that it is diagnostic for CADASIL (7, 8).
5 Cell Culture Models: Testing the Molecular Functions of CADASIL Alleles
Joutel and colleagues modified a cloned human NOTCH3 gene, to create the equivalent of five known CADASIL mutations, which were assayed in cell culture (transient transfection) assays: R90C and C212S (located in EGF repeats 2–5), C428S (located in the ligand-binding domain, EGF repeats 10–11), C542Y (EGF repeat 13), and R1006C (EGF repeat 26). Their results showed that all five mutant receptors reached the cell surface in the normally cleaved form (131). Only the C542Y mutant had a significantly reduced number of receptors at the cell surface. Only the C428S mutant lost its Jagged1-binding ability. Both C542Y and C428S exhibited a net significant reduction in ligand-induced reporter gene expression (Notch signaling), but the other three mutant receptors (R90C, C212S, R1006C) appeared to retain normal levels of Notch signaling ability (131). One difficulty with these experiments was that transient transfection experiments create a cell population in which individual cells have varying gene copy numbers, which tends to reduce the ability to detect small changes in gene activity. However, Joutel et al. were able to simulate “heterozygotes” by transfecting with equal amounts of wild-type and mutant NOTCH3 genes, and these experiments confirmed dose-dependent (intermediate) levels of Notch signaling in C428S/+ and C542Y/+ “heterozygotes,” providing convincing evidence that Notch signaling by these alleles was significantly different from the R90C, C212S, and R1006C alleles (131). Human patients who were heterozygous for three of these mutations (R90C, C212S, C428S) were compared to each other histologically, and found to have similar amounts of arterial aggregates of the NOTCH3 extracellular domain, indicating that the accumulation of GOM did not require ligand binding by the NOTCH3 receptor (131).
A similar study was conducted by Kalaria and colleagues (132), who genetically engineered the cloned human NOTCH3 gene to create four different mutations (R90C, R133C, C185R, and R449C). These were overexpressed under control of the CMV promoter, by stable transfection in two different cell lines (HEK293 and SH-SY5Y). The rates of processing (assayed by pulse-chase analysis), transport to the cell surface (estimated by immunofluorescence), and Notch signaling (assayed by reporter gene expression) were not significantly different from control experiments, in which the normal NOTCH3 gene was similarly overexpressed (132).
< div class='tao-gold-member'>
Only gold members can continue reading. Log In or Register a > to continue
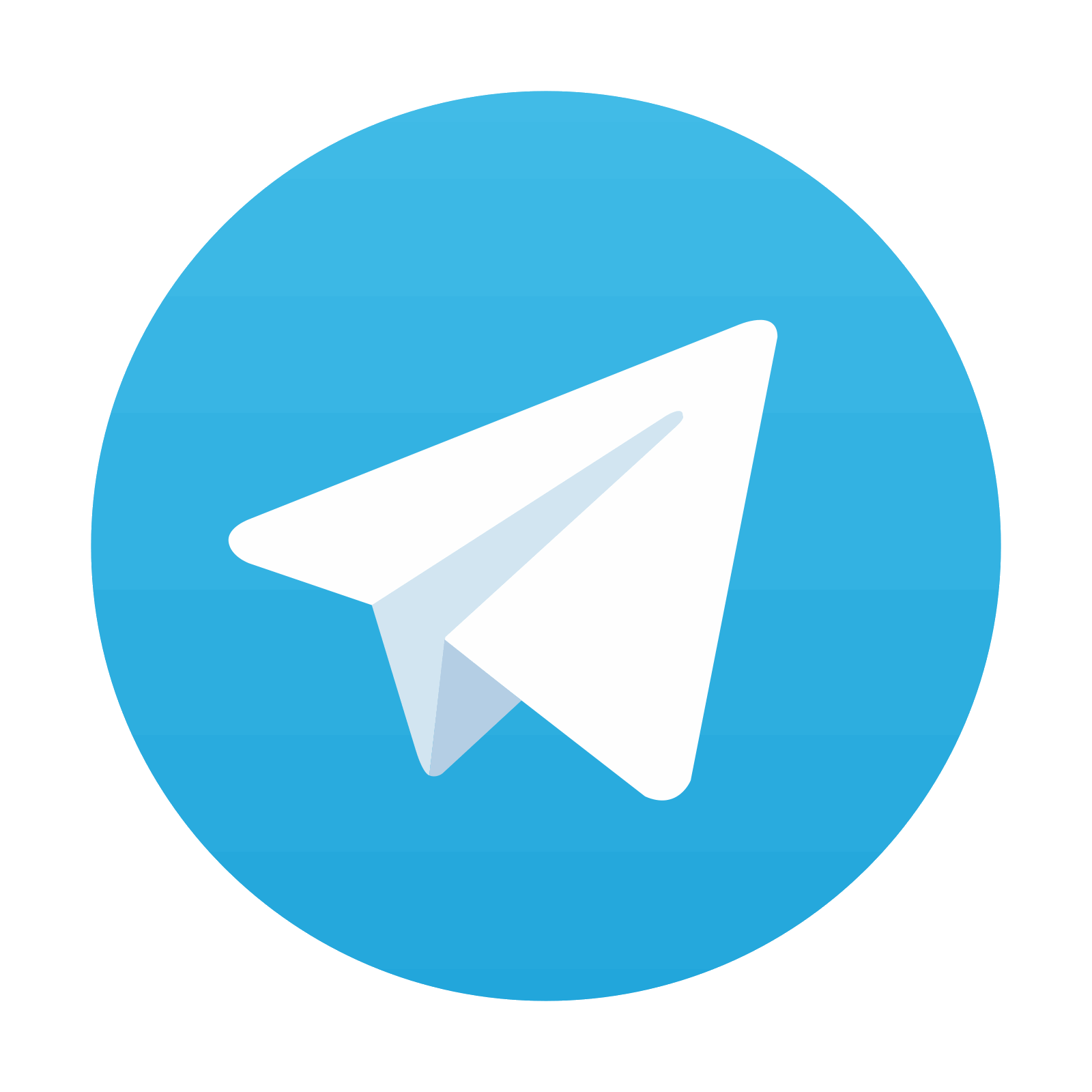
Stay updated, free articles. Join our Telegram channel
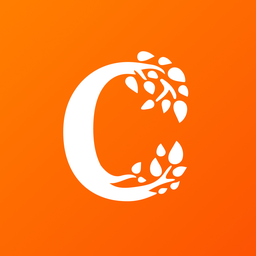
Full access? Get Clinical Tree
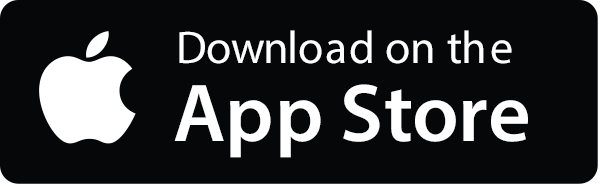
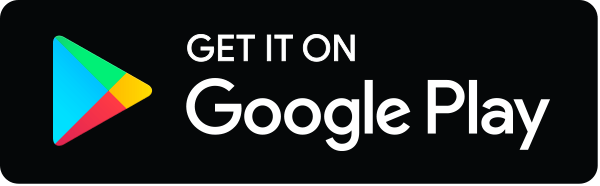