Chapter 28 Avian Clinical Biochemistry II. COLLECTION OF BLOOD SAMPLES III. STARVATION, FLIGHT, AND POSTPRANDIAL EFFECTS: CIRCADIAN AND CIRCANNUAL RHYTHMS B. Biochemistry of Long-Term Starvation C. Biochemistry of Endurance Flight C. Physiological Variation IN Female Birds D. Refractometry versus the Biuret Method E. Effect of Protein Standards F. Plasma Protein Electrophoresis: Albumin/Globulin Ratio A. End Products of Protein Metabalism: Hyperuricemia and Gout B. Articular and Visceral Gout C. Acute versus Chronic Renal Failure G. Other Changes Associated with Renal Failure B. Enzyme Activities in Avian Tissues C. Clearance of Enzymes from Plasma D. Experimentally Induced Liver and Muscle Disease H. Plasma Ammonia: Hepatoencephalopathy K. Clinical Diagnosis of Liver Disease VIII. CALCIUM AND PHOSPHORUS: METABOLIC BONE DISEASE A. Relation between Calcium and Protein in Avian Plasma C. Physiological Marrow Ossification D. Hypocalcemia Syndrome in African Grey Parrots E. Alkaline Phosphatase in Bone Disease IX. DIABETES MELLITUS AND PLASMA GLUCOSE X. EXOCRINE PANCREATIC DISEASE C. Organophosphate and Carbamate Avian medicine and surgery have been recognized as an official specialty in veterinary medicine on three continents (Europe, Australia, and North America). The increasing demand for veterinary care for individual birds with a high sentimental or economical value and efforts to conserve endangered species facilitated this awareness. The commercial poultry flock approach to diagnosis, utilizing necropsy as a primary diagnostic tool combined with comparatively fewer testing procedures on living birds, which had been practiced for decades, had only limited applicability for individual pet birds. As a result, alternative diagnostic and therapeutic techniques were developed. Clinical signs in birds are often nonspecific, and the information gained by physical examination is limited in regard to specific and detailed diagnosis. Earlier demands for large blood sample volumes and limited veterinary involvement in the diagnosis and management of individual and pet bird disease were major obstacles to the development of clinical biochemistry in avian medicine. The introduction of micromethods in clinical laboratories and the public demand for veterinary care for individual birds have removed these obstacles. The scientific and clinical work in avian clinical biochemistry since the 1980s has led to its widespread application in avian medicine. Reference values are dependent on the methodology used. Factors such as type of coagulant, amount of blood, and analytical method may all influence the results. Therefore, values from clinical cases should be compared with reference values from the same species established with the same method in the same laboratory. Published reference values for many blood chemical variables can only be used as a rough guideline. All efforts should be made to obtain a blood sample before any treatment is given. Treatments that have been administered before samples have been collected may severely affect plasma chemical values (see Fig. 28-16), which will jeopardize a correct diagnosis at a later stage. The time interval between restraint and blood sampling should be kept to a minimum to prevent stress-associated changes in clinical chemistry parameters (see Section VII). Paradoxically, blood samples should be obtained before an extensive clinical examination has been performed to avoid iatrogenic changes in the samples. In one study with pigeons, the percentage of heterophils more than doubled whereas the percentage of lymphocytes decreased after extensive handling for 3h. Creatine kinase and glucose both increased, whereas uric acid decreased (Scope et al., 2002b). Although the changes associated with a short clinical examination might be negligible, the clinician should keep these iatrogenic effects in mind when performing more extensive procedures. An important consideration when taking blood samples from small birds is response to blood loss. Kovàch et al. (1969) studied the mortality of various avian and mammalian species following blood loss and showed that birds can better tolerate severe blood loss than mammals (Fig. 28-1). This is because of their greater capacity for extravascular fluid mobilization (Djojosugito et al., 1968; Wyse and Nickerson, 1971). Kovàch et al. (1969) found that in healthy individuals, the amount of blood that can be removed without deleterious effects is 3% of body weight in ducks and pigeons, 2% in chickens, and 1% in crows and pheasants (Fig. 28-1). Unless birds are severely debilitated, a maximum of 1% seems a safe limit for the amount of blood that can be collected for diagnostic purposes. FIGURE 28-1 Mortality after identical blood losses in various avian and mammalian species (abscissa). Every hour, 1% of body weight blood was withdrawn from every animal (ordinate). The percentage of animals lost during the hour following bleeding has been recorded and plotted (see Kovàch et al., 1969). Reprinted with permission from Lumeij (1987a). Nearly all routine hematological and biochemical investigations can be performed when lithium heparin is used. The use of one single sample limits unnecessary blood spillage, which is an important consideration when dealing with small birds. When plasma is used instead of serum, more plasma can be harvested than serum from the collecting tube. Another reason for not using serum in avian samples is the risk of clotting of the supernatant when serum and cells are separated within a couple of hours after collection. In mammals, EDTA is regarded as the best anticoagulant for preservation of cellular morphology and good staining characteristics (Schmidt et al., 1963), but this is not necessarily true in hematology of all avian species. There are various avian species where EDTA causes disruption of the red blood cells. Hawkey et al. (1983) found that EDTA produced progressive hemolysis in blood samples from crowned cranes. Dein (1986a, 1986b) reported a similar reaction in crows, jays, brush turkey, and hornbills. Similar reactions to EDTA are observed in blood from crows and magpies (Lumeij, unpublished). Fourie (1977) found heparin to be the most suitable anticoagulant for hematology in pigeons. Good quality smears can also be obtained from whole blood without anticoagulants. Whatever method is used, blood smears should be made immediately after collection of the sample to prevent changes in blood cell morphology. The normal time lag of up to 60min between collection of a blood sample and separation of plasma from cells, which is common in human medicine (Laessig et al., 1976), is not acceptable in avian clinical biochemistry. Immediately after collection, plasma and cells should be separated by centrifuging. In pigeon blood at room temperature, a rapid decline (10% in 10 min, 30% in 30 min, up to 65% in 2 h) of plasma potassium concentrations occurs, because of a shift of potassium ions from the plasma into the red blood cells. In chickens, decreases were smaller overall with a 29% decrease being noted after 2 h (Lumeij, 1985a). In ostriches, significant increases in plasma potassium concentrations were observed when blood was stored at 20°C (up to 20% in 2 h), whereas at 0°C significant decreases were observed (Verstappen et al., 2002). Many reports on blood chemistry in birds are based on determinations in serum instead of plasma or plasma from blood samples that were not centrifuged immediately. Plasma potassium concentrations reported herein are often too low. In suspected lead poisoning, heparinized whole blood samples should be sent to the laboratory, because the majority of lead is associated with the red blood cells (see Section XI.A). In most species, the right jugular vein is the preferred site for blood sampling. This thick walled vein is less prone to hematoma formation (Law, 1960; McClure and Cedeno, 1955; Stevens and Ridgeway 1966). The medial metatarsal vein is especially useful for multiple sampling of small blood volumes in larger birds such as pigeons. Blood can be collected using a needle and syringe or a blood lancet. In the pigeon, the jugular vein is not readily visible. The basilic vein, which is readily visible as it crosses the ventral aspect of the elbow of all avian species, is the vein that is traditionally used in poultry. The vein is punctured with a blood lancet after being swabbed with alcohol (Gratzl und Koehler 1968). These authors warn against the use of the comb for blood collection in poultry because the high risk of exsanguination, especially during cold weather. In pet birds, the use of a blood lancet for blood collection from the basilic vein cannot be recommended because this site is prone to hematoma formation, often even when a needle is used. The advantage of the basilic vein, on the other hand, is that it can be located in all avian species. In ostriches the operator should be aware of the risk of being kicked. Blood can be collected from the basilic vein using a sideway approach to the standing animal after it has been hooded and the wing has been lifted upward by two assistants (Fowler, 1978c). The jugular vein can also be approached in the same manner. In ducks and geese, the venous occipital sinus is a good site for blood sampling (Vuillaume, 1983). It is located at the junction of the base of the skull and the first cervical vertebra. Although this site is especially useful for obtaining large samples, many clinicians will feel more comfortable using the easily accessible basilic and metatarsal veins in these species. Cardiac puncture carries the risk of cardiac tamponade, and therefore this technique is not recommended for use in avian clinical practice. Some individuals choose to clip a toenail to obtain a blood sample. Disadvantages of this method are that it is painful to the bird, the sample may become contaminated with tissue fluids, it may cause damage to the nail bed, and the amount of blood that can be obtained is limited. Furthermore, contamination of the sample with urates from the droppings may give false high readings (Ekstrom and Degernes, 1989; Rosskopf et al., 1982). For the aforementioned reasons, this method should only be regarded as a last resort, and the nail should be thoroughly cleaned before obtaining a sample. Different bleeding sites (e.g., venous blood versus blood collected by cardiac puncture) may cause variation in hematological or biochemical values (Kern and De Graw, 1978). A vacuum system greatly facilitates blood sampling from the jugular and basilic veins and from the venous occipital sinus in Anseriformes. A 3-ml vacuum tube is sufficient for most cases (Venoject, Omnilabo, Breda, The Netherlands). For smaller birds, and thus smaller sample sizes, it is best to use small volume (e.g., 0.5 ml) Vacutainers (Veterinary Lab Supply, 315 E. Madison, Winterset, Iowa 50273, United States). Some plasma chemical variables are influenced by starvation or food consumption. Up to 4 days of starvation in pigeons did not result in hypoglycemia, but rather a starvation hyperglycemia occurred after 3 days (Lumeij, 1987b). Variables that may have markedly increased values postprandially are uric acid and total bile acid concentrations. See Sections V.F. and VI.G (Lumeij, 1991; Lumeij and Remple, 1991, 1992). Furthermore, daily or yearly fluctuations have also been reported for some chemical variables. In fasted pigeons maintained on a natural daily 17-h photoperiod a circadian rhythm was found in plasma glucose concentrations (Lumeij et al., 1987b) with high values early during the photophase (Fig. 28-2). Basal plasma thyroxine concentrations in racing pigeons were significantly higher in July than in September and December (Lumeij and Westerhof, 1988a). Age, sex, altitude, nutritional status, and egg laying may also cause variation (Driver, 1981; Kocan, 1972; Kocan and Pits, 1976; McGrath, 1971; Mori and George, 1978; Simkiss, 1967). Effects of long-term starvation and endurance flight are discussed in Sections III.B. and III.C, respectively. Many avian species depend on catabolism of lipid depots for survival through the night or winter to enable migratory flights or to incubate eggs (Blem, 2000). During migratory flights, energy expenditure may be more than seven times the basic metabolic rate for 3 to 5 days (Battley, 2003). Lipid reserves in birds are stored as triglycerides, which have a high caloric density and do not require water. Storage of fat reserves may double the body mass in some cases. FIGURE 28-2 Mean ( ± SEM) plasma glucose concentration as a function of time in fasted racing pigeons. A cosine function y(t) is fitted to the data: y(t) = 16.26 + 1.55 • cos (0.2618t to 2.4646). The relevance of the fit as judged by means of the multiple correlation coefficient was significant (R = 0.892, p<0.01). Reprinted with permission from Lumeij et al. (1987b). Starved birds go through three phases. The total duration of the various phases depends on the size of the bird and the amount of lipid reserves and may vary from a few hours in hummingbirds to 5 months in emperor penguins (Robin et al., 1998). In phase 1, food in the digestive tract and glycogen reserves are used; in phase 2, lipids are metabolized; and in phase 3, protein is used as a substrate for glucose synthesis. Phase 2 of starvation is characterized by an almost constant rate of body mass loss, a low respiratory quotient, and steady plasma concentrations of uric acid and β hydroxybutyrate. Although fatty acids provide the main energy source, about 5% of the energy is provided by protein breakdown, to generate citric acid intermediates, to act as substrate for gluconeogenesis, and for production of antioxidants (Battley, 2003). When critical depletion of fat stores is imminent, phase 3 of starvation is heralded by lowering of plasma concentrations of β-hydroxybutyrate and increased uric acid concentrations, reflecting a decreased contribution of lipids to energy metabolism and increased protein catabolism, respectively (Fig. 28-3). The refeeding drive is related to the attainment to a given energy status rather than to a given duration of fasting or body mass loss (Robin et al., 1998). The metabolic shift to an increased protein breakdown is regulated by an endocrine shift (elevated corticosterone concentration), after which a further adrenocortical response to an acute stressor is inhibited. The adrenocortical response typical for an emergency situation is only reached when muscle protein is dangerously low (Jenni et al., 2000). FIGURE 28-3 Changes in specific daily body mass, plasma uric acid, corticosterone, and β-hydroxybutyrate (β-OHB), and behavior versus body mass in spontaneously fasted emperor penguins (X ± SE; n = 6). Crosshatched bars represent periods of display songs. The behavioral index was calculated as number of days an animal was active during successive periods of fasting corresponding to a 2-kg loss in body mass. Reprinted with permission from Robin et al. (1998). Mortality resulting from hepatic lipidosis has been described in a wide variety of avian species (James et al., 2000; Wadsworth et al., 1984) including, among others, chickens (Butler, 1976), turkeys (Gazdinski et al., 1994), parrots (Baker, 1980; Murphey, 1992a), raptors (Cooper and Forbes, 1983; Forbes and Cooper, 1993), and bustards (Nichols et al., 1997) and is known as fatty liver syndrome. Although the exact mechanism has not been elucidated, it seems that deficiencies of other nutrients, which are essential in lipid metabolism, like the amino acids methionine and cysteine and the vitamin biotin, may play a crucial role in the pathophysiology of this syndrome (Butler, 1976). Because of the lack of these essential components for lipid metabolism, a buildup of lipids occurs in the liver, which eventually leads to liver failure. The need to conserve body protein during starvation has been stressed in extremely obese persons who were treated by starvation, because slow loss of protein during complete starvation may lead to sudden death because of a cumulative protein loss (Le Maho et al., 1988). From a physiological point of view, birds thus seem to be well equipped to deal with prolonged periods of starvation through prolonged metabolism of fat as the major energy source, provided they have sufficient fat stores and sufficient essential amino acid and vitamin stores to facilitate lipid catabolism. When clinically monitoring obese birds during a forced starvation period, plasma concentrations of corticosterone, β-hydroxybutyrate and uric acid can be used to pinpoint the critical transition from phase 2 to phase 3 of starvation. When starving obese birds, which have a history of malnutrition, to force them to change over to a balanced diet, it seems prudent to give a multivitamin injection and small amounts of a mixture of essential amino acids to avoid a deficiency of lipotrophic factors and starvation-related hepatic lipidosis. After a 90- to 160-min flight of 48 km, homing pigeons show marked changes in plasma chemistry, which include increased glucagon like immunoreactivity (GLI), increased concentrations of free fatty acids (FFA) and triglyceride (TG), decreased thyroxine (T4), triiodothyronine (T3), and T3/T4 ratio (George et al., 1989). Viswanathan et al. (1987, 1988) observed significant increases in plasma glucose and lactate, FFA, and growth hormone (GH), but not corticosterone after a 80- to 90-min flight of 48 km. In contrast to George et al. (1989), they did not see changes in T4 and T3. George et al. (1992) documented under similar conditions a significant increase of plasma arginine vasotocine (AVT) without change in plasma osmolality. However, in free-flying tippler pigeons trained to fly continuously for up to 5 h, Giladi et al. (1997) found three- to eightfold increased plasma AVT (up to 100 pg/ml), increased plasma osmolality and decreased hematocrit values. Bordel and Haase (1993, 2000) studied the influence of flight duration on blood parameters in homing pigeons that returned after 2 to 22 h from release sites 113 to 620 km away. Hematocrit values decreased from 54% in controls to 51% in flown birds. Plasma FFA levels increased significantly during flight, and TG concentrations gradually decreased with progressive flight duration. Plasma concentrations of glucose and lactate did not differ between experimental and control birds. Immediately after takeoff and up to ≈5 h of flight, plasma uric acid (UA) increased in a linear manner and reached values of 1500 μmol/after flight duration >5 h to 22 h (two to fourfold increase of control values), whereas urea (UR) levels gradually rose with flight duration to 400% of control values. Plasma protein decreased in flown pigeons. The excretion of UR, uric acid and Nτ-methylhistidine was significantly higher in flown birds compared to controls during 1 to 3 days immediately following return, but immediately after flight Nτ-methylhistidine did not elevate. These findings support the view that lipids are the main energy source during flight. The increase in lactate during short flights is compatible with the idea that carbohydrates are utilized as fuel mainly in the initial phase of flight and are used for the activity of the white glycolytic fibers in the flight muscles. Furthermore, protein catabolism increases during endurance flights. Because UR formation in pigeons occurs mainly through arginolysis (Bordel and Haase, 1998) and increased protein breakdown raises the availability of arginine (Robin et al., 1987), the elevated plasma concentrations of UR and UA can be attributed to an accelerated protein breakdown during flight. The increased availability of free amino acids and their conversion into metabolites of the citric acid cycle could enhance the capacity of the tricarboxylic acid cycle and thereupon the oxidation of acetyl-CoA derived from lipolysis (Dohm et al., 1985). In addition, protein degradation contributes to the prevention of dehydration during flight because the catabolism of a mixture of 70% lipids and 30% protein yields ≈20% more water than the catabolism of pure fat (Klaasen, 1996). Because the methylated amino acid Nτ-methylhistidine occurs almost exclusive in actin and myosin filaments and is excreted after myofilament breakdown, the findings suggest an increased breakdown of myofibrillar proteins in the immediate period after the flight, probably as a result of repair processes of contractile elements in the muscles as a reaction to protein breakdown during flight (Bordel and Haase, 2000). The AVT increase can be regarded as an overall homeostatic mechanism during homing flights, whereby (1) lipid is mobilized, (2) water is conserved, and (3) temperature is regulated. There is a significant correlation between postflight AVT and body mass loss (which in flying birds represents mainly water loss). Water loss is related to the duration of flight and the environmental temperature. Despite substantial water loss, the hematocrit of flying pigeons significantly decreases. This probably results from expansion of plasma volume through a shift of water from the interstitial fluid. The expanded plasma volume and reduced hematocrit may contribute to the maintenance of blood pressure, whereas the decreased hematocrit may enhance blood flow to metabolically active tissues. FIGURE 28-4 Relation between plasma and serum total protein by biuret method in racing pigeons (n = 50; g/l). From Lumeij and Maclean (1996). FIGURE 28-5 Relation between plasma total protein concentration by biuret method and two types of refractometers in pigeons (g/L; n = 58). Symbols: •, temperature compensated refractometer (y = –16.38 + 1.93 X; r = 0.89); о, nontemperature compensated refractometer (y = –25.88 + 2.06 X; r = 0.89). From Lumeij and McLean (1996). The absence of changes in plasma corticosterone concentrations during the shorter flights can be considered as an absence of stress under the circumstances studied. Plasma proteins are important complementary constituents in the diagnosis of gastrointestinal, hepatic, renal, or infectious diseases. Determination of plasma proteins seldom leads to a specific diagnosis (e.g., monoclonal gammopathies) but will help the clinician to evaluate the nature, severity, and progress of a disease. In pigeons, the concentration of total protein (TP) in plasma is about 1.5 g/L higher than in serum, because the former also contains fibrinogen: TPserum = –1.7 + 1.01 TPplasma (Fig. 28-4). According to Lumeij and McLean (1996), the correlation is highly significant (p < 0.000001; R = 0.99; n = 50). Differences between plasma and serum were discussed in Section II.B. When plasma rather than serum is used, recognition of elevated fibrinogen concentration can be seen in the protein electrophoresis and is reflected by elevation of the acute phase (α or β) proteins (Roman et al., 2005). See also Section XII.C.6. In female birds, a considerable increase in plasma total protein concentration occurs just before egg laying because of an estrogen-induced increase in the globulin fractions (Griminger, 1976). The proteins are the yolk precursors (e.g., vitellogenin and lipoproteins), which are synthesized in the liver and transported to the ovary, where they are incorporated in the oocyte (Griffin et al., 1984). Lumeij and De Bruijne (1985b) demonstrated that the refractometric method is unreliable for use in avian blood and therefore this method should not be used in avian practice. The refractometric method consistently yields higher values when compared to total protein concentrations determined with the biuret method, and the correlation coefficient between these two methods is low. One study suggested that only temperature compensated refractometers are reliable (Andreasen et al., 1989). In another study in our laboratory (Lumeij and McLean, 1996), using plasma and serum of 58 pigeons, two types of refractometers were compared with the biuret method. Neither instrument proved to give an accurate measurement of plasma total protein. Both refractometers gave considerably higher values than the biuret method, with the temperature compensated instrument being consistently higher in readings than the nontemperature compensated one (Fig. 28-5). It was concluded that a species- and refractometer-specific conversion factor must be applied before refractometric values can be used, and then only as a rough estimate of the TP. Another important consideration is that TP determinations (refractometric or by biuret method) without information on plasma protein electrophoresis have limited value (see later discussion). For avian clinical practice, it is advised that the clinician establish TP values using the biuret method. Most commercial laboratories use a human standard for TP and albumin (Alb) determinations, without validating the method for the species of which the blood sample is submitted. There are significant differences between TP concentrations when different standards are used (e.g., human, bovine, pigeon, and chicken standards), although there is a high correlation between the results obtained with the various standards (Lumeij et al., 1990; Spano et al., 1987,1988). When a pigeon standard was used to determine serum TP concentration (TPp) with the biuret method, values found were significantly higher compared to values found determined with the biuret method using the human standard (TPh), but there was a high correlation (Lumeij et al., 1990): Spano et al. (1987, 1988) found consistently lower TP values in chicken serum using a chicken standard compared with a bovine standard. Because the use of a species-specific standard for all species presented to the avian practitioner is unrealistic and because a high correlation exists between the results obtained with the various standards, it is recommended that clinicians establish reference values for the various species using the standard that is most commonly used in commercial laboratories (i.e., the human standard). Plasma protein electrophoresis (PPE) on cellulose acetate membranes has been widely used in avian patients (Lumeij, 1987e; Lumeij and De Bruijne, 1985a). In many laboratories, agarose gel films are replacing cellulose acetate membranes. A good correlation (r = 0.998) exists between these methods in human serum (Archer and Battison, 1997). Protein fractions that can be observed include Alb, α, β, and γ globulin. The α and β globulins (including fibrinogen) are considered acute phase proteins, whereas the γ fraction is elevated in chronic conditions and includes the immunoglobulins. Often a prealbumin fraction can also be observed (Fig. 28-6). In healthy birds, the Alb fraction is the largest protein fraction. In acute or chronic inflammatory conditions, a rise in total protein caused by elevated globulin fractions may occur. Often Alb concentrations are decreased in these situations. The combined effect of these changes is a decrease in the Alb/Globulin (A/G) ratio. Often the total protein concentration is within the reference range, whereas the A/G ratio is decreased, therefore the A/G ratio is of greater clinical significance than the total protein concentration. Examples of diseases with a decrease in the A/G ratio are egg related peritonitis and chronic infectious diseases such as aspergillosis, psittacosis, and tuberculosis. In ducks, Liu et al. (1984) found that serum globulin increased with the severity of pathological changes of amyloidosis and that the globulin was also found in the amyloidotic tissue. Protein electrophoresis can also be used to monitor response to treatment. In liver failure, extremely low plasma protein concentrations can occur in combination with a decreased A/G ratio. Gastrointestinal and renal diseases can also lead to severe hypoproteinemia. In birds, protein malnutrition may lead to hypoproteinemia (Leveille and Sauberlich, 1961). Increased TP concentrations with a normal A/G ratio can be expected in dehydrated birds if the primary disease did not cause hypoproteinemia. To calculate the A/G ratio, prealbumin and Alb as determined by plasma protein electrophoresis are combined as “A” and all globulin fractions as “G” (Figs 28-8 through 28-11; Lumeij, 1987e). FIGURE 28-6 Densitometer scan and electrophoretic pattern on a cellulose acetate membrane from a representative pigeon serum. Prealbumin, albumin, α, β, and γ globulin. Reprinted with permission from Lumeij and De Bruijne (1985a). In plasma of some species, the mobility of Alb in cellulose acetate and agarose gels is less compared to the usual patterns, as seen, for example, in chickens and pigeons. In the cockatiel, for example, prealbumin migrates to a position equivalent to chicken albumin, and albumin to a position equivalent to chicken γ globulins (Fig. 28-7). Tatum et al. (2000) has confirmed the diagnostic value of PPE for a wide variety of raptor species. Rosenthal et al. (2005a) questioned the reliability of PPE in birds. However, they limited their study to healthy birds, and their data did not span the full range of clinical values. Because values of individual globulin fractions in healthy birds are low and boundaries between the various globulin fractions are not clear, it is to be expected that large bias is introduced when an arbitrary (manual) distinction between the various globulin fractions has to be made (Cray, 2005; Rosenthal et al., 2005b). Their study did, however, confirm the high reliability of determinations of TP and Alb, whereas measurement of γ-globulins had fair to good agreement. Determination of the A/G ratio as described earlier and visual inspection of the globulin fractions in case of abnormal values will leave no doubt on the fractions responsible for the abnormalities, as, for example, in the hyperglobulinemia reported by De Wit et al. (2003). FIGURE 28-7 Agarose gel electrophoresis. From left to right, chicken (Gallus gallus domesticus) serum (lane 1), purified chicken albumin (lane 2), chicken plasma (lane 3), cockatiel (Nymphicus hollandicus) plasma (lane 4), purified cockatiel albumin (lane 5), and cockatiel serum (lane 6). Chicken albumin (lanes 1, 2, and 3, band b) migrated further than cockatiel albumin (lanes 4 and 6, band a). Cockatiel albumin migration was similar to that of chicken α globulins (lanes 1 and 3, band a), whereas cockatiel prealbumin migration (lanes 4 and 6, broad bands b) was similar to that of chicken albumin. Purification of cockatiel albumin (lane 5, single band) altered its migration pattern, whereas purification of chicken albumin (lane 2, single band b) did not have this effect. Reprinted with permission from Archer and Battison (1997). The labor-intensive PPE is not available in every laboratory, and Alb is commonly determined chemically by the bromcresol green (BCG) dye-binding method. The BCG method is unreliable in avian blood. Discrepancies between values obtained by dye-binding techniques and those obtained by electrophoresis have been demonstrated for chicken, duck, turkey, and pigeon (Lumeij et al., 1990; Spano et al., 1987, 1988). In general, Alb determinations performed with dry methods have not been validated for use in birds. Furthermore, various Alb standards can be used in different laboratories, although most commercial laboratories will use a human standard for TP and Alb determinations. The method used should have been validated for the species in question and compared with reference values established in the same laboratory. FIGURE 28-8 Plasma protein electrophoresis and the albumin:globulin ratio (A/G) in an African grey parrot (Psittacus erithacus erithacus) with psittacosis, before, during, and after treatment with doxycycline. Symbols: Pre, prealbumin; alb, albumin; α, β, γ, globulin fractions (reference values in parentheses). Reprinted with permission from Lumeij (1987e). Prealbumin is the most rapidly migrating fraction in avian plasma and has been associated with binding thyroxine and retinol (thyroxine binding prealbumin-TBPA; transthyretin). Although the protein travels anodal to albumin in birds, primates, and the horse, it is usually not visualized in the last. In other species transthyretin travels cathodal to albumin or has the same motility to albumin, which explains the absence of a “prealbumin” fraction in these species using routine electrophoresis techniques (Chang et al., 1999; Larsson et al., 1985). Seasonally high concentrations of plasma transthyretin concentrations (150 to 200 mg/L in May through July versus 80 to 100 mg/L in September through January) have been associated with molting in storks (Cookson et al., 1988). FIGURE 28-9 Plasma protein electrophoresis in an Amazon parrot (Amazona sp.) with aspergillosis. At 4½ months after a diagnosis was made and despite treatment with ketoconazole and 5-fluorocytosine, the globulin fraction was still elevated, causing the A/G ratio to decrease (reference values in parentheses). Reprinted with permission from Lumeij (1987e). FIGURE 28-10 Serum protein electrophoresis and albumin:globulin (A/G) ratio in an Emu (Dromiceius novaehollandiae) with an egg-related peritonitis. Two months after surgical treatment and remission of clinical signs, a marked increase in the albumin fraction and decrease of the globulin fraction was observed. Reprinted with permission from Lumeij (1987e). FIGURE 28-11 Total protein concentration, plasma protein electrophoresis, albumin:globulin ratio (A/G), and plasma aspartate aminotransferase (AST) activity in an Amazon parrot (Amazona sp.). At the first examination there was a marked elevation of AST and decreased A/G. Liver cirrhosis was diagnosed by means of histological examination of a liver biopsy. The second protein electrophoresis was made from a plasma sample collected just before the bird was euthanized 1 month later. Reference values in parentheses. Reprinted with permission from Lumeij (1987e). Uric acid (UA) is the major end product of nitrogen (N) metabolism in birds. It constitutes approximately 60% to 80% of the total excreted N in avian urine (Skadhauge, 1981). The formation of urea (UR) in pigeons occurs mainly through arginolysis (Bordel and Haase, 1998). Uricotelism permits excretion or storage of N waste in a small volume of water. UA is relatively nontoxic when compared to UR or ammonia (NH3), which is essential for the development of the embryo in the egg of reptiles and birds. UA is synthesized in the liver, and 90% is excreted via tubular secretion, largely independent of urine flow rate (Skadhauge, 1981). The clearance of UA exceeds the glomerular filtration rate by a factor 8 to 16. The rate of secretion is largely independent of the state of hydration. Very high concentrations of UA can be found in ureteral urine in dehydrated birds. Renal function disorders can eventually lead to elevated plasma UA concentrations. Nonprotein nitrogen (NPN) substances in plasma such as UA, creatinine (Cr), and UR will only be elevated when renal function is below 30% of its original capacity. For elevated UA and UR during starvation and endurance flight see Sections III.B and III.C. Hyperuricemia can result in precipitation of monosodium urate monohydrate (MSUM) crystals in joints (articular gout) and on visceral surfaces (visceral gout). The exact mechanism of deposition or the predilection for certain sites is unknown, although lower temperatures at predilection sites have been suggested. Gout should not be regarded as a disease but as a clinical sign of any severe renal function disorder. When birds are provided with dietary protein in excess of their requirements, the surplus protein is catabolized and the N released converted to UA. The total amount of UA formed may surpass the clearing capacity of this substance from the body and hyperuricemia, and articular gout may result. The use of high-protein poultry pellets as the bulk food in psittacines may result in an increased incidence of gout. There is no consensus on the different etiologies of articular and visceral gout in birds. The following hypothesis, however, seems to explain all known facts about avian gout. A plasma UA concentration that is slightly above the solubility of MSUM will lead to UA precipitates in the body. Predilection sites are those areas where the solubility of MSUM is lower than in other areas. The joints and synovial sheaths may be predilection sites because of a comparatively low temperature. Articular gout is a sign of chronic moderate hyperuricemia. MSUM deposits grow with time with chronic hyperuricemia and form the typical tophi of articular gout. If urates precipitate in the tubules or collecting ducts of the kidney or the ureters (e.g., severe dehydration of long duration, vitamin A deficiency), this will lead to an acute obstructive uropathy (postrenal obstruction). Anuria or gross oliguria and tubular secretion of UA are severely compromised. This leads to a rapid and severe increase in plasma UA with precipitation of urate crystals on many visceral surfaces and those predilection sites for articular gout. This condition of visceral gout will rapidly lead to death of the affected animal. This hypothesis is based on the fact that no inflammation or tophi are seen in typical predilection sites for articular gout, because the condition has a rapidly fatal course. There is no time for an inflammatory reaction or tophi to develop. In this situation, the kidney tubules, collecting ducts, and ureters may contain UA deposits. An alternative situation could occur in acute tubular failure. In this condition, visceral gout could develop without UA deposits in the tubules, collecting ducts, and ureters. Renal function disorders may result from any progressive destructive condition affecting both kidneys (chronic renal failure), or from conditions wherein the function of the kidneys is rapidly and severely, but often reversibly, compromised (acute renal failure). In the latter condition, oliguria is usual, whereas in the former situation, polyuria is normally observed. It is important to differentiate between reversible conditions (e.g., prerenal renal failure caused by dehydration or shock of any cause, urolithiasis [postrenal renal failure] and acute nephritis) and chronic irreversible renal failure. Appropriate and timely treatment of acute renal failure can often prevent further damage and in some cases result in improved function. Extrarenal factors such as infection, gastrointestinal hemorrhage, and hypovolemia can disturb an otherwise stable, well-compensated asymptomatic chronic renal patient and precipitate a desperately dangerous condition. Prerenal azotemia can be defined as the clinical condition associated with reduced renal arterial tension leading to oliguria and retention of nitrogenous waste products in the blood. It is often seen during shock or severe dehydration. No increased plasma UA concentrations were observed in 4-day dehydrated racing pigeons, whereas plasma UR concentration had a significant 6.5- to 15.3-fold increase. Plasma UR appeared to be the single most useful variable for early detection of prerenal causes of renal failure (Lumeij, 1987c). This is because UR is excreted by glomerular filtration, whereas tubular reabsorption is dependent on urine flow, which in turn depends on the state of hydration. During hydration, almost all of the filtered UR is excreted and during dehydration nearly all of the filtered UR is reabsorbed. The active tubular secretion of UA, on the other hand, is not dependent on arterial pressure, because the tubules of the reptilian type nephrons are supplied by venous blood through the renal portal system. The tubular reabsorption of UR in conditions of renal failure accompanied by a low urine flow (e.g., dehydration) in combination with a nearly unchanged tubular secretion of UA causes a disproportionate increase in plasma UR concentration, resulting in an elevated UR/uric acid ratio. Although potentially useful for judging the hydration status of a bird, UR is normally present in low concentration in avian plasma and has traditionally been considered an inappropriate variable to evaluate renal function in birds. When the dehydration becomes more severe, this may eventually lead to hyperuricemia. This might be caused by reduced tubular blood supply which leads to reduced uric acid secretion. Urates may also precipitate in the tubules when there is active tubular secretion of UA in the absence of urine flow. The latter condition looks much like acute uric acid nephropathy in humans (Watts, 1978). There is a great deal of confusion with respect to the conversion of urea (CH4N2O) to blood UR nitrogen or “BUN.” Apart from the fact that BUN only refers to the nitrogen part of the UR molecule, in the United States it is often expressed in mg/dl, whereas in Europe UR is usually expressed in SI units (mmol/L). It is sad to see how reference values that were presented in the previous edition of this book were erroneously converted to American units in the Journal of Veterinary Clinical Pathology (Harr, 2002). To convert BUN in mg/dl to urea in mmol/L, the following steps must be performed: 1. Convert BUN (mg/dl) to urea (mg/dl) by multiplying BUN with the quotient of the molecular mass of urea and the molecular mass of nitrogen in the urea molecule (12 + 4 × 1 + 2 × 14 + 16)/(2 × 14). 2. Convert urea (mg/dl) to urea (mmol/L) by dividing urea (mg/dl) by the molecular mass of urea (60). 3. Convert mg/dl to mg/L by multiplying the result by 10. Or more simply: to convert BUN (mg/dl) to urea (mmol/L), divide BUN with total mass of nitrogen in urea molecule and multiply by 10 (10/28) = 0.357). The conversion from UR (mmol/L) to BUN (mg/dl) is 1/0.357 = 2.8. In raptors, a significant postprandial increase in plasma UA and UR concentrations occurs (Lumeij and Remple, 1991, 1992). Postprandial UA was similar to that in birds with hyperuricemia and gout and was well above the theoretical limit of solubility of urate in plasma. It is not clear why under physiological conditions, no urate deposits occur in raptors, which have hyperuricemia for at least 12 h after ingesting a natural meal (Fig. 28-12). A similar effect was noted in the piscivorous blackfooted penguin (Spenicus demersus) (Kolmstetter and Ramsay, 2000). To avoid physiological food-induced elevations in UA and UR that can complicate interpretation of plasma chemistry results in raptorial or piscivorous birds, blood samples should ideally be collected after a 24-h fasting period. In small passerines, this is obviously not an option! FIGURE 28-12 Fasting and postprandial nonprotein nitrogen substances in plasma of peregrine falcons, Falco peregrinus (mean ± SD). Symbols: Hyperkalemia is a particular problem in acute renal failure that may lead to severe electrocardiographic changes and eventually to cardiac arrest. Hypocalcemia and hyperphosphatemia are usual in humans with renal failure. The former may lead to hypocalcemic tetany, especially with rapid correction of acidosis. In birds, special attention should be paid to these variables for further documentation of changes in renal disease because these changes may have therapeutic implications. Anemia has been documented in birds with chronic renal failure. Macroscopically, the aspirated urates from articular gout look like toothpaste. The presence of urate can be confirmed by performing the murexide test or by microscopic examination of aspirates of tophi or joint accumulations. The murexide test is performed by mixing a drop of nitric acid with a small amount of the suspected material on a slide. The material is evaporated to dryness in a Bunsen flame and allowed to cool. Then one drop of concentrated ammonia is added. If urates are present, a mauve color will develop. Microscopically sharp needle-shaped crystals about the size of a leukocyte can be seen in smears of joint fluid from patients with articular gout. A polarizing microscope is helpful in identifying the typical birefringent crystals. Birefringent literally means splitting a ray of light in two. Crystals bend light and become visible in joint fluid when viewed through a microscope with crossed polarizing filters. When a compensator plate is used on the microscope, monosodium urate crystals parallel to the axis of the compensator appear yellow (negatively birefringent). In humans, pseudo-gout is diagnosed by finding positively birefringent calcium pyrophosphate dihydrate crystals that appear blue when parallel to the axis of the compensator. Calcium pyrophosphate dihydrate crystals are smaller and are rhomboid shaped. Clinical enzymology is described more fully in a separate chapter in this book, so only a brief synopsis is given here. Enzymes occur normally in the cytoplasm (e.g., aspartate aminotransferase [AST], alanine aminotransferase [ALT], lactic dehydrogenase [LDH]), mitochondria (glutamate dehydrogenase [GLDH] and AST), nucleus, or membranes (alkaline phosphatase [AP], gamma glutamyl transferase [GGT, γGT]) of body cells, where they catalyze specific reactions. The distribution of various enzymes is markedly different among organs and animal species, which explains the variation in organ and tissue specificities among animal species. Generally, increased plasma enzyme concentrations indicate recent organ damage rather then decreased organ function. Increased enzyme production has been reported in cholestatic liver disease in mammals (AP and GGT). Sometimes a decreased activity is of diagnostic value (e.g., decreased cholinesterase activity in organophosphate toxicity). Baseline activity of an enzyme in plasma is generally a reflection of the amount and turnover of the tissue that contains this enzyme. For example, the creatine kinase (CK) activity in plasma increases in direct proportion to the increase of skeletal muscle mass as a result of training. Increases in CK may also be observed simply as a result of the muscle cellular damage associated with capture and restraint. Conversely, in chronic liver diseases with severe fibrosis and a reduction in the number of functional hepatocytes, plasma activities of liver enzymes may be within normal limits. The increase of a particular enzyme also depends on factors such as its rate of release, rate of production, and rate of clearance from plasma. Cytoplasmatic enzymes will be released early in cell degeneration, whereas mitochondrial enzymes will be released after advanced cell damage (necrosis). Enzymes with high tissue concentrations but with short elimination half-lives are of limited value in clinical enzymology because of their rapid disappearance from plasma. Generally, EDTA samples are not appropriate for enzyme assays, because this anticoagulant may chelate metal ions, which are required for maximal enzyme activity. Plasma and cells should be separated immediately after sampling to prevent leakage of intracellular enzymes into the plasma. Even if the cellular elements are separated from the plasma, freezing/thawing and refrigeration of plasma samples for several days may severely decrease enzyme activity and therefore should be avoided unless the effects of the storage procedure used is known. Enzyme profiles of the various organs have been studied in chickens, mallards, turkeys, racing pigeons, budgerigars and African grey parrots (Lumeij 1994d; Figs. 28-13 through 28-15). The half-life of an enzyme is defined as the time required for its concentration to be reduced by half. When an enzyme is injected into plasma, its clearance from the plasma generally follows a biphasic exponential decline. Initially, there is a rapid decline, which is the primary mixing or distribution phase, followed by a slower secondary decline phase, which is the actual clearance of the enzyme from the plasma. During this secondary phase, a constant fraction of enzyme present is cleared per unit of time; hence, the decline is linear on a semilogarithmic scale. The (t½β)half-life of the enzyme can be calculated from the regression function of the secondary linear phase of the semilogarithmic concentration-time curve and is independent of plasma enzyme activity. Clearance half-lives of various enzymes considered to be of use for the differential diagnosis of liver and muscle disease in pigeons have been established by studying the disappearance rates of enzymes from plasma after IV injections of supernatants of homologous liver and muscle homogenates (Lumeij et al., 1988a). For AST, ALT, and LD, the half-lives of the respective enzymes from liver and muscle were compared, whereas for GLDH and CK, only liver and muscle were used, respectively (Table 28-1). Plasma enzyme profiles after experimentally or spontaneously occurring liver disease have been studied in a number of avian species. The results of studies in racing pigeons (Lumeij et al., 1988a, 1988b) with two different types of liver disease were compared to plasma chemistry changes after muscle injury. Liver disease was induced by ethylene glycol or D-galactosamine, and muscle injury was induced by an intramuscular injection of doxycycline in three groups of six pigeons each. Plasma chemical changes were correlated with histological findings from organ samples taken just after the last blood collection (Fig. 28-16, Table 28-2). Plasma AST activity and bile acid (BA) concentration were the most sensitive indicators of liver disease in the racing pigeon, followed by ALT, GGT, and LD. Although all pigeons with histological proven ethylene glycol- or galactosamine-induced liver damage had increased AST activity and BA concentrations in their plasmas, these constituents were not raised at every sampling time. Increased plasma GLDH activities were associated with large necrotic areas in the liver. Moderate necrosis of liver cells resulted in slightly elevated GLDH activities. Degeneration of liver cells and hepatitis with single-cell necrosis did not give rise to elevated plasma GLDH activities. Plasma CK activity was never increased because of liver damage, whereas GLDH, GGT, and BA were never elevated during muscle damage. Thus, these four constituents are useful for differentiating between liver and muscle disease. GLDH is the most liver-specific enzyme in the racing pigeon. Because GLDH is localized within the mitochondria of the liver cells, increased plasma GLDH activities, however, are only observed after liver cell necrosis. FIGURE 28-13 Relative distribution of various tissue enzymes in supernatants of pigeon tissue homogenates. Mean ± SEM, U/g wet tissue, n = 6. Reprinted with permission from Lumeij et al. (1988a). FIGURE 28-14 Relative distribution of various tissue enzymes in supernatants of tissue homogenates from the budgerigar, Melopsittacus undulatus. Mean ± SEM, n = 7, U/g wet tissue. Reprinted with permission from Lumeij and Wolfswinkel (1987). FIGURE 28-15 Relative distribution of various tissue enzymes in supernatants of tissue homogenates from the African grey parrot, Psittacus erithacus. Mean ± SEM, U/g wet tissue. Reprinted with permission from Lumeij (1994d).
I. INTRODUCTION
II. COLLECTION OF BLOOD SAMPLES
A. Size of Blood Samples
B. Handling of Blood Samples
C. Sampling Procedure
III. STARVATION, FLIGHT, AND POSTPRANDIAL EFFECTS: CIRCADIAN AND CIRCANNUAL RHYTHMS
A. Introduction
B. Biochemistry of Long-Term Starvation
C. Biochemistry of Endurance Flight
IV. PLASMA PROTEINS
A. Introduction
B. Plasma versus Serum
C. Physiological Variation in Female Birds
D. Refractometry versus the Biuret Method
E. Effect of Protein Standards
F. Plasma Protein Electrophoresis: Albumin/Globulin Ratio
G. Albumin Methodology
H. Prealbumin
V. RENAL FUNCTION
A. End Products of Protein Metabolism: Hyperuricemia and Gout
B. Articular and Visceral Gout
C. Acute versus Chronic Renal Failure
D. Prerenal Azotemia
E. Urea versus BUN
F. Postprandial Effects
, [uric acid] (μmol/L); Δ, [urea] (mmol/L); O, [creatinine] (μmol/L); ↓, feeding quail. Reprinted with permission from Lumeij and Remple (1991).
G. Other Changes Associated with Renal Failure
H. Murexide Test
I. Birefringent Crystals
VI. HEPATOBILIARY DISEASE
A. Clinical Enzymology
B. Enzyme Activities in Avian Tissues
C. Clearance of Enzymes from Plasma
D. Experimentally Induced Liver and Muscle Disease
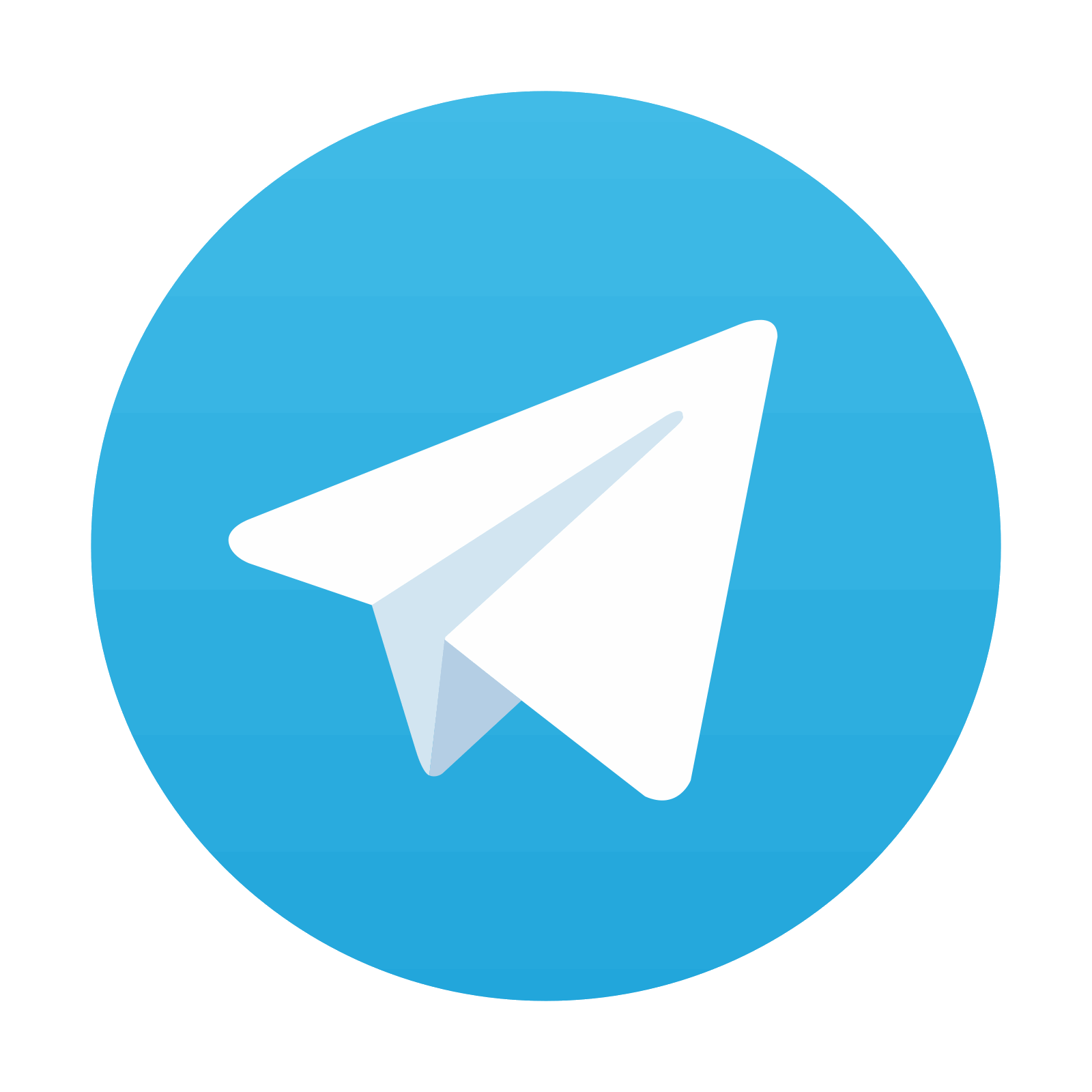
Stay updated, free articles. Join our Telegram channel
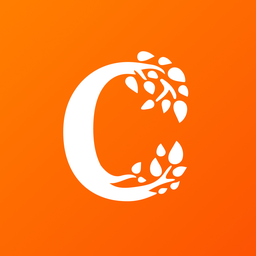
Full access? Get Clinical Tree
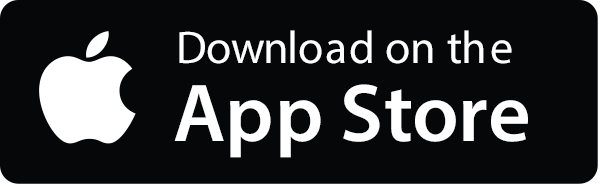
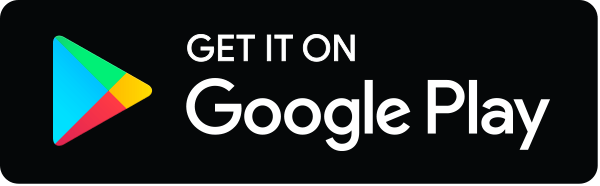