Chapter 2 Applied Renal Physiology
Each day the glomeruli of the kidneys filter an enormous volume of plasma water, and the tubules must reabsorb most of this water along with vital solutes so that only a small volume of water and unneeded solutes are excreted as urine. For example, a normal 10-kg dog may have a glomerular filtration rate (GFR) of 4 mL/min/kg. In the course of one day, this dog would filter 57.6 L of plasma water in its kidneys. If 60% of body weight is water, this volume represents almost 10 times the dog’s total body water. The same dog may have a urine output of 33 mL/kg/day. Thus, more than 99% of plasma water filtered by the glomeruli is reabsorbed by the tubules. The proximal tubules and loops of Henle reabsorb approximately 85% of the filtered water and solutes, whereas the collecting ducts adjust the final composition of urine to compensate for fluctuations in intake and prevent changes in the volume and composition of body fluids. The major functions of the various segments of the nephron are depicted in Figure 2-1.
Glomerular filtration
Glomerular morphology
The glomerular capillary wall or filtration barrier consists of three components: the capillary endothelium, basement membrane, and visceral epithelium (Fig. 2-2). The glomerulus is a unique vascular structure consisting of a capillary bed interposed between two arterioles: the afferent and efferent arterioles. The glomerular capillary divides into several branches, each of which forms a lobule of the glomerulus. The capillary endothelium of the glomerulus is fenestrated by openings 50 to 100 nm in diameter. These openings exclude cells from the ultrafiltrate, but macromolecules are not restricted based on size. The luminal surface of the endothelium is covered by negatively charged sialoglycoproteins that contribute to the charge selectivity of the filtration barrier.
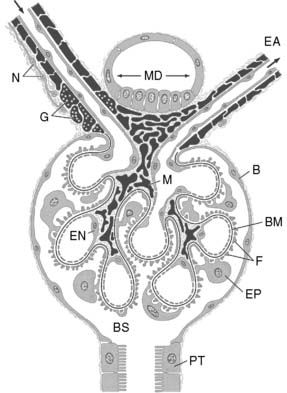
Figure 2-2 Schematic representation of the glomerulus demonstrating the afferent and efferent arterioles, juxtaglomerular apparatus, and glomerular capillary loops. At the vascular pole, an afferent arteriole (AA) enters and an efferent arteriole (EA) leaves the glomerulus. At the urinary pole, the Bowman space (BS) becomes the tubular lumen of the proximal tubule (PT). The epithelial cells composing the Bowman capsule (B) enclose the Bowman space. Smooth muscle cells proper of the arterioles and all cells derived from smooth muscle are shown in black, including the granular cells (G).The afferent arteriole is innervated by sympathetic nerve terminals (N). The extraglomerular mesangial cells are located at the angle between AA and EA and continue into the mesangial cells (M) of the glomerular tuft. The glomerular capillaries are outlined by fenestrated endothelial cells (EN) and covered from the outside by the epithelial cells (EP) with foot processes (F). The glomerular basement membrane (BM) is continuous throughout the glomerulus. At the vascular pole, the thick ascending limb touches the macula densa (MD), the extraglomerular mesangium.31
The charge selectivity of the glomerulus resides in the negatively charged sialoglycoproteins (e.g., laminin and fibronectin) and peptidoglycans (e.g., heparan sulfate) of the capillary endothelium, lamina rara interna, lamina rara externa, and visceral epithelium. At any given effective molecular radius, negatively charged macromolecules experience greater restriction to filtration than neutral ones. Positively charged macromolecules experience less restriction to filtration than neutral ones of the same size (Fig. 2-3).
Determinants of glomerular filtration
These relationships are depicted in Figure 2-4, in which average pressure values are those reported for dogs36 and cats.7 If the average pressures just described are considered alone, it can be seen that the net filtration pressure in the glomerulus is approximately 15 mm Hg, which is similar to values obtained for systemic capillaries. The fact that GFR is so much higher than the movement of fluid across systemic capillaries is explained by different values for Kf.
Changes in the resistance of the afferent (preglomerular) and efferent (postglomerular) arterioles may have a marked effect on GFR. Alterations in resistance in the afferent arterioles lead to parallel changes in GFR and renal blood flow (RBF), but changes in resistance in the efferent arterioles lead to divergent changes in GFR and RBF (Fig. 2-5). The interplay of the effects of neural and hormonal factors on vascular tone in the kidneys is complex, but the main purpose of these effects is to minimize even slight changes in GFR, which could have drastic adverse effects on the volume and composition of the extracellular fluid.
The resistance of these arterioles is regulated by the autonomic nervous system and by numerous vasoactive mediators (Table 2-1). Stimulation of the sympathetic nervous system results in release of norepinephrine from nerves terminating on the afferent and efferent arterioles. Norepinephrine can cause afferent and efferent vasoconstriction, but efferent arteriolar constriction usually predominates. As a result, RBF decreases with minimal changes in GFR (i.e., filtration fraction [FF] increases). Angiotensin II also causes efferent more than afferent vasoconstriction and has similar effects on RBF and GFR. Stimulation of dopaminergic receptors causes afferent and efferent vasodilatation and increased RBF with little change in GFR at low concentrations of dopamine. Norepinephrine, angiotensin II, and antidiuretic hormone (ADH, vasopressin) cause vasoconstriction, at the same time promoting the production of prostaglandins that cause vasodilatation. These prostaglandins (PGE2 and PGI2) play an important role in maintaining RBF in hypovolemic states when angiotensin II and norepinephrine concentrations are increased. The effects of these prostaglandins are limited to the kidneys because they are rapidly metabolized in the pulmonary circulation. Nonsteroidal anti-inflammatory drugs that inhibit generation of prostaglandins by the cyclooxygenase pathway may cause renal ischemia and acute renal insufficiency in hypovolemic patients.10,12 Locally produced kinins also cause vasodilatation and favor redistribution of RBF to inner cortical nephrons. Mediators produced locally by the vascular endothelium also contribute to afferent and efferent vasoconstriction (e.g., endothelin and thromboxane) and vasodilatation (e.g., nitric oxide and prostacyclin).
Table 2-1 Effects of Selected Vasoactive Mediators on Glomerular Hemodynamics
Substance | Afferent Arteriole | Efferent Arteriole |
---|---|---|
Vasodilators | ||
Acetylcholine | Relax | Relax |
Nitric oxide | Relax | Relax |
Dopamine | Relax | Relax |
Bradykinin | Relax | Relax |
Prostacyclin | Relax | Relax |
Prostaglandin E2 | Relax | No effect |
Prostaglandin I2 | Relax | Relax |
Vasoconstrictors | ||
Norepinephrine | Constrict | Constrict |
Angiotensin II | Constrict | Constrict |
Endothelin | Constrict | Constrict |
Thromboxane | Constrict | Constrict |
Vasopressin | No effect | Constrict |
From Valtin H, Schafer JA. Renal function. Boston: Little, Brown, 1995: 107.
Measurement of glomerular filtration rate
Note that this equation is the same as the formula for clearance presented before. Thus, the renal clearance of a substance that is neither reabsorbed nor secreted is equal to GFR. Inulin is a polymer of fructose with a molecular mass of 5200 da. It is not bound to plasma proteins and is freely filtered by the glomeruli. It is neither reabsorbed nor secreted by the tubules. It is not metabolized by the kidneys or any other organ. It is uncharged and not subject to the Gibbs-Donnan effect. In summary, inulin is an ideal substance for the measurement of GFR, and inulin clearance is the laboratory standard for GFR determination. Normal values for GFR as measured by inulin clearance are 3 to 5 mL/min/kg in the dog16,21 and 2.5 to 3.5 mL/min/kg in the cat.16,45
In the dog and cat, creatinine is filtered by the glomeruli and is neither reabsorbed nor secreted by the tubules.18–20,22 In most clinical pathology laboratories, creatinine is measured by the alkaline picrate reaction. This reaction is not entirely specific for creatinine and measures another group of substances collectively known as noncreatinine chromogens. These substances are found in plasma, where they may constitute up to 50% of the measured creatinine at normal serum creatinine concentrations, but only small amounts appear in urine.21,22 When the creatinine concentration is determined using the alkaline picrate reaction, the presence of noncreatinine chromogens causes endogenous creatinine clearance to underestimate GFR. This problem may be avoided by using more accurate methods (e.g., peroxidase-antiperoxidase) to measure the creatinine concentration.22 Values for endogenous creatinine clearance in the dog and cat are approximately 2 to 5 mL/min/kg.5,17,22
To circumvent the problem of noncreatinine chromogens and to improve accuracy, some investigators have advocated determination of exogenous creatinine clearance. In this test, which is somewhat more cumbersome, creatinine is administered subcutaneously to the animal to increase the serum creatinine concentration and reduce the relative effect of the noncreatinine chromogens. For example, a normal dog may have a serum creatinine concentration of 1.0 mg/dL, of which 0.5 mg/dL represents noncreatinine chromogens. This measurement represents a 50% error. If, however, the dog’s serum creatinine concentration is increased to 10 mg/dL by subcutaneous administration of creatinine, the noncreatinine chromogens still represent only 0.5 mg/dL, and the error is reduced to 5%. Exogenous creatinine clearance exceeds endogenous creatinine clearance and more closely approximates inulin clearance in the dog.20
where Tx is the amount handled by tubules (milligrams per minute).
Renal blood flow and renal plasma flow
The kidneys receive 25% or more of cardiac output. The major sites of resistance within the kidneys are the afferent and efferent arterioles, with an approximately 80% to 90% decrease in perfusion pressure across this region of the renal vasculature (Fig. 2-6). Blood flow is not uniform throughout the kidneys. In dogs, more than 90% of RBF is normally directed to the renal cortex, less than 10% to the outer medulla, and only 2% to 3% to the inner medulla.51 The actual rate of flow to the renal cortex is approximately 100 times that of resting muscle and is required for glomerular filtration. Blood flow to the medulla is similar to that of resting muscle, and this reduced flow is necessary for normal function of the urinary concentrating mechanism.
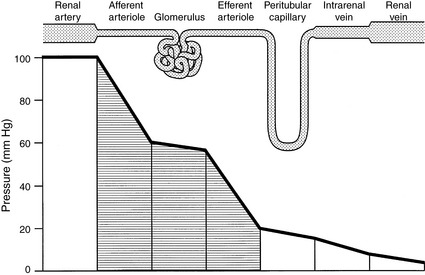
Figure 2-6 Pattern of hydrostatic pressure and vascular resistance in the renal circulation.
(Drawing by Tim Vojt.)
Autoregulation
Autoregulation refers to the intrinsic ability of an organ to maintain blood flow at a nearly constant rate despite changes in arterial perfusion pressure. In the kidneys, between perfusion pressures of 80 and 180 mm Hg, GFR and RBF vary less than 10% (Fig. 2-7). Flow (Q) is equal to pressure (P) divided by resistance (R). As pressure increases, flow can remain constant only if resistance increases proportionately. The site of this resistance change in the kidneys is the afferent arteriole. Autoregulation is intrinsic to the kidneys and occurs in the isolated, denervated kidney and in the adrenalectomized animal. However, it is impaired by anesthesia in proportion to the depth of anesthesia. The afferent arterioles are maximally dilated at mean arterial pressures of 70 to 80 mm Hg, and at lower pressures, GFR declines linearly with RBF (i.e., autoregulation is lost). It is likely that autoregulation of RBF is a consequence of the need to regulate GFR closely and thus maintain tight control over water and salt balance.Two physiologic mechanisms contribute to autoregulation. The myogenic mechanism is based on the principle that smooth muscle tends to contract when stretched and relax when shortened. As the afferent arteriole is stretched by increased perfusion pressure, it constricts, thus limiting transmission of this increased pressure to the glomerulus and minimizing any change in glomerular capillary hydrostatic pressure and SNGFR. The myogenic mechanism represents a coarse control that operates with a delay of 1 to 2 seconds.
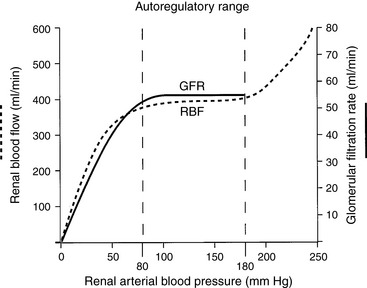
Figure 2-7 Autoregulation of renal blood flow and glomerular filtration rate.
(Drawing by Tim Vojt.)
Tubuloglomerular feedback represents a local intrarenal negative feedback mechanism for individual nephrons. The morphologic basis for this physiologic mechanism is the JGA. Increased sodium chloride concentration or transport in the distal tubule is sensed by the extraglomerular mesangial cells of the JGA as they monitor sodium chloride transport across the tubular cells of the macula densa. Transport of NaCl by the tubular cells of the macula densa requires functional NKCC2 (the Na+, K+, 2Cl– cotransporter) and ROMK (a potassium channel) in the luminal membranes and functional Na+, K+-ATPase in the basolateral membranes.46 Transcellular transport of NaCl causes generation of adenosine, which together with angiotensin II causes afferent arteriolar constriction in the parent glomerulus. The afferent arteriolar constriction causes SNGFR to decrease, thus decreasing filtration and minimizing NaCl loss in that nephron. This effect occurs locally in the region of the juxtaglomerular interstitium. Tubuloglomerular feedback represents a fine control that operates with a 10- to 12-second delay.
Measurement of renal blood flow and renal plasma flow
Consider the following mass balance equation52:
Amount entering the kidneys = amount leaving the kidney
A more accurate calculation of RPF is then:
Note that this equation is identical to that derived before using the mass balance principle.
If the hematocrit is known, RBF can be calculated from the RPF by using the following equation:
In the dog and cat, normal values for RPF are 7 to 20 mL/min/kg and 8 to 22 mL/min/kg, respectively.38,39,45
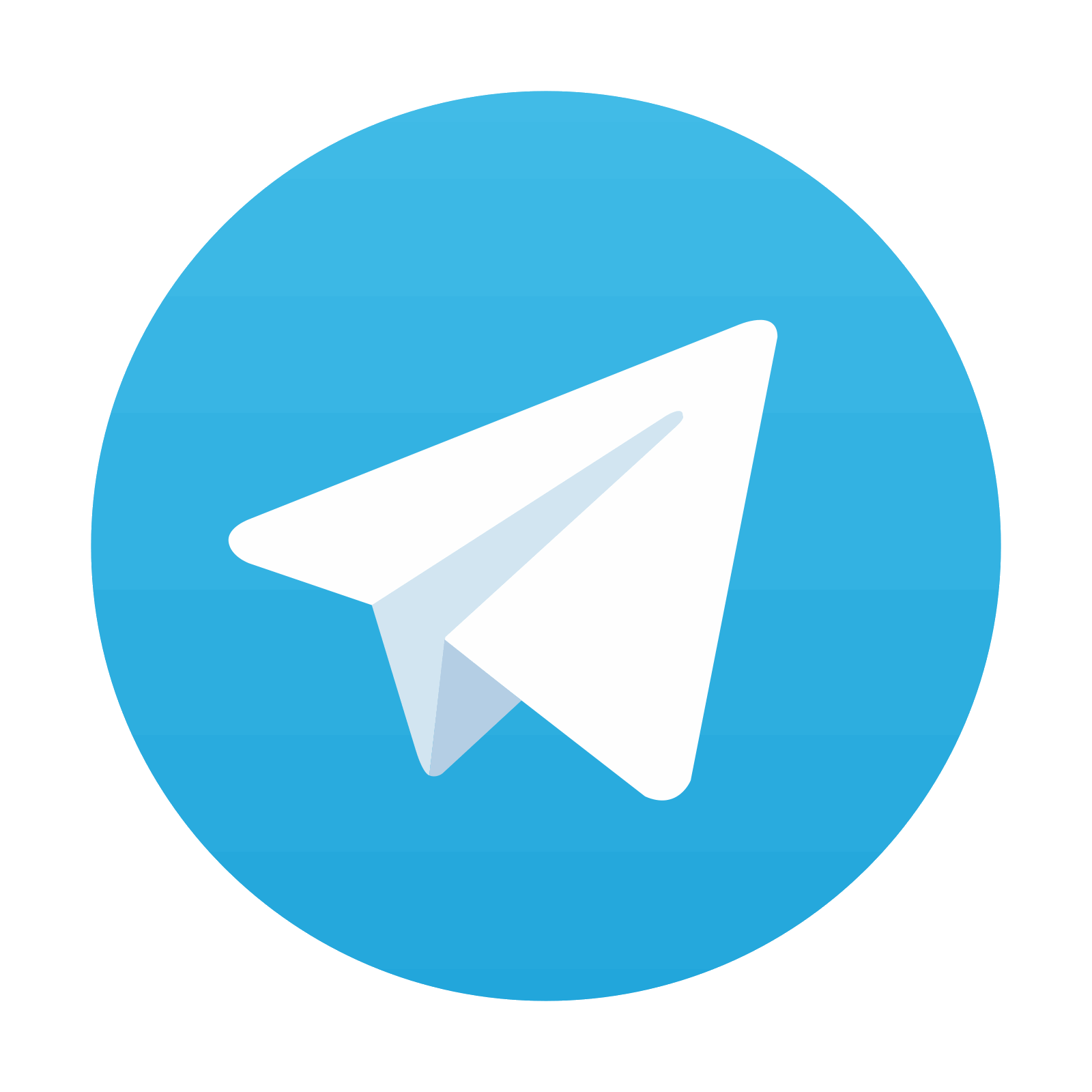
Stay updated, free articles. Join our Telegram channel
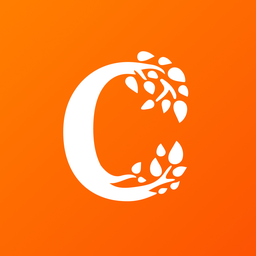
Full access? Get Clinical Tree
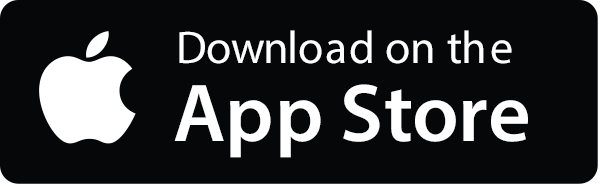
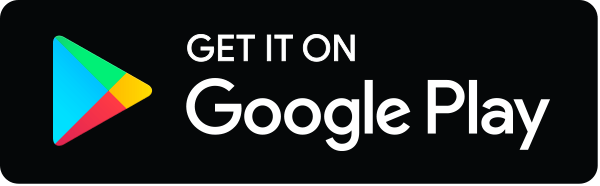