(1)
Division of Human Anatomy, Department of Biomedical Sciences and Biotechnology, Faculty of Medicine, University of Brescia, Brescia, Italy
Abstract
Alzheimer’s disease (AD) is nowadays the most widespread form of senile dementia in Western countries. AD is considered a multifactorial disease: in fact, it is probable that many concomitant factors (genetic and environmental) are relevant in the pathogenesis of this disease. In particular, among the environmental factors, a lot of recent interest has been raised concerning the possible involvement of metal dyshomeostasis. Several studies have indicated the imbalance of many trace elements in AD, including aluminum (Al), iron (Fe), lead (Pb), manganese (Mn), copper (Cu), and zinc (Zn). We have therefore analyzed the role of these metal ions in AD development.
Key words
Animal modelsADMetalsOxidative stress1 Introduction
Several million people worldwide are affected by neurodegenerative diseases, a heterogeneous group of diseases affecting specific areas of the central nervous system (CNS). These can lead to gradual and progressive cognitive or movement impairment, depending on the type of neuronal cells undergoing selective degeneration (1). Alzheimer’s disease (AD) is nowadays the most widespread form of senile dementia in Western countries. It is clinically characterized by gradual onset with progressive and irreversible cognitive decline. Memory impairment appears in the earliest stage of the disease while patients’ motor and sensory functions are usually not affected until later stages (2). It is almost impossible to give an in vivo differential diagnosis between AD and normal aging. Indeed, only postmortem microscopic examination reveals the critical features of AD, i.e. accumulation of insoluble β-amyloid (Aβ) peptides in extracellular senile plaques and deposition of misfolded microtubule-associated tau protein in neurofibrillary tangles within neuronal bodies (3). Both these neuropathological hallmarks cause nerve cell death and so damage the functions of the CNS. Nowadays, the etiology of AD remains unknown. It is probable that many concomitant factors are relevant in AD pathogenesis. So we can consider AD as a multifactorial disease. AD is genetically complex: rare mutations in three genes (amyloid precursor protein (APP), and presenilins-1 and -2) are responsible for cases of familial early-onset (<65 years) autosomal dominant forms (1). Sporadic forms of AD are most probably due to the contribution of several susceptibility genes at multiple loci and their interaction with environmental factors. The major risk factor (other than aging) for the development of AD is a genetic polymorphism in the gene for ApoE, a lipoprotein involved in synaptic repair and in maintenance of neuronal structure (4). Individuals who carry one copy of the APOE-ε4 allele have a two- to threefold increased risk of AD, compared to the more common APOE-ε3 allele. However, the APOE-ε2 allele seems to give some protection (5). The finding that monozygotic twins do not necessarily both develop AD (6), clearly suggests that there is also an exogenous contribution related to diet, lifestyle, and the patient’s anamnesis (7).
Among environmental factors, aging remains the major risk factor for AD in the general population. An interesting hypothesis states that free radicals produced during cellular respiration could play an important role both in the process of aging and in the development of AD (8). Another predisponent factor for AD is the occurrence of cerebral infarcts, whose incidence increases with aging (9). Moreover, several epidemiological studies have shown that head injury is also a risk factor (10) because it seems to be associated with the formation of neurofibrillary tangles (11) and Aβ deposition (12). Recent studies suggest that AD is an immune disorder from blood brain barrier (BBB) disruption (13), characterized by selective antibodies for cholinergic neurons. Even if there is no evidence due to the vicinity of rinencephalon and certain regions of the CNS involved in AD neurodegeneration, many authors have proposed a link between amyloid deposition and viral pathogens that enter the nervous system through olfactory receptors (14). At present, many authors accept that the immune system is involved in the etiology of AD. This premise is founded on the numerous alterations in AD brains that show the activation of immunological and inflammatory mechanisms. From an environmental point of view, a lot of recent interest has been raised concerning the possible involvement of metal dyshomeostasis in AD pathogenesis. We have therefore analyzed the role of metal ions in AD development.
2 Metals Dyshomeostasis and Oxidative Stress
The CNS appears to be particularly vulnerable to oxidative stress. A number of factors contribute to the relatively high vulnerability of the CNS to oxidative damage. These include low levels of the natural antioxidant glutathione present in neurons (15), a high proportion of polyunsaturated fatty acids contained in membranes (16), and a very high oxygen requirement due to the high metabolic activities of the brain (17). Oxidative stress is accompanied in varying degrees by a dyshomeostasis of metal ions, including redox-active transition metals (copper, iron, and manganese) as well as redox inactive metal ions such as zinc. Trace redox-active transition metals are essential for most biological reactions (e.g., in the synthesis of DNA, RNA, and proteins) and as cofactors for numerous enzymes, particularly those involved in respiration. Their deficiency can therefore lead to disturbances in CNS function. Accumulations of redox-active transition metals in tissues are cytotoxic due to their participation in many cellular disturbances characterized by increased free radical production (18). Mounting evidence indicates that dyshomeostasis of cerebral metals and APP/Aβ/metal redox interactions may contribute to the neuropathology of AD (19). Characteristic of AD is a dyshomeostasis of both redox-active and redox-inactive metal ions. Several studies have indicated the imbalance of many trace elements in AD, including aluminum (Al), lead (Pb), zinc (Zn), copper (Cu), manganese (Mn), and iron (Fe), with only the latter three being redox-active. Redox-inactive metal ions may be pathogenic by virtue of their ability to impair the metabolism of redox-active metal ions. Moreover, they may also contribute to neurodegeneration through their deleterious effects on protein and peptide structures, including for example pathological aggregation phenomena. Microparticle-induced X-ray emission data have revealed that insoluble Aβ plaques in postmortem AD brains have abnormally enriched Al, Cu, Fe, and Zn (20). Evidence for the imbalance of trace metal homeostasis in AD have led to the identification of possible interactions of metal ions with both APP and Aβ. Metals such as Al, Fe, Zn, and Cu in vitro promote Aβ aggregation in plaques (21–23). The finding that metal chelators can at least partially solubilize Aβ deposits and attenuate the cerebral Aβ burden in APP transgenic mouse models of AD (24, 25) suggests their possible therapeutical use (26, 27). Interestingly, a more recent study indicates that coprecipitant ions (such as Zn(II)) may be needed for in vivo Aβ aggregation since the Aβ1–40 peptide is thermodynamically soluble at physiological concentrations without any factors (28). Moreover, the 50-untranslated region (50UTR) of APP mRNA has a functional iron-response element (IRE) for the bond with Fe (29), which is consistent with a role for APP as a redox-active metalloprotein (30). Some pilot studies also indicate that Fe, Cu, and Zn ions are able to promote APP expression linking its 50UTR in a dose-dependent manner. Cerebral biometal metabolism is probably intimately associated with APP processing. Again, the gene expression profile of AD brain implicates dysregulation of cerebral metal metabolism (31). Compared to controls, gene expression levels for metal regulatory proteins such as metallothionein III and metal regulatory factor-1 decreased more than fourfold in AD brain (32). In addition to oxidative damage to proteins, oxidative stress also causes oxidative damage to nucleic acids, in particular RNA. 8-Hydroxyguanosine is a nucleic acid oxidation marker commonly observed in the cytoplasm of those neurons particularly vulnerable to degeneration in AD (33). There is always the question of whether increased tissue burden of metals reflects at least partial dietary factors (e.g., there is mixed evidence for a role of dietary Cu in experimental models of AD). The brain normally concentrates Cu, Fe, and Zn in the neocortex (20). Environmental metal exposure has also been suggested to be a risk factor for AD. The most studied ones are Al and Fe (34, 35), while evidence for Pb, Mn, Cu, and Zn is sparse. Clearly, the complex biochemical relation between biometal metabolism and environmental metal exposure and AD pathophysiology warrants further study. All these findings are consistent with the refined amyloid cascade hypothesis (36). However, it is not known whether metal excess causes oxidative stress and neurodegeneration or whether metal excess is a consequence of neuronal cell loss.
3 Experimental Models of Metallotoxicity
Many experimental approaches can be used to study the potential metal involvement in the neuropathogenesis of AD. In particular, in vivo studies can be very useful to establish whether a metal is toxic for the CNS and if it can induce or facilitate the development of AD-like lesions. For the general design of an in vivo experiment, a large number of parameters have to be considered, including animal species, age of animals, and metal exposure routes (7). Many recent findings concerning metal-induced AD have been obtained using transgenic animals (e.g., AD mice or mice models with accelerated aging) combined with metal exposure. Studies on aged animals, or indeed studies with long-term exposure over months or years, are therefore better reflections of the human situation, where metals build-up (especially for nonacute toxicity ones) occurs over a long time. To date, a variety of in vivo procedures are available to assess the consequences of long-term exposure to metals. These comprise a range of behavioral tests, but also in vivo electrophysiological procedures (both in anesthetized and freely moving animals) and in vivo brain microdialysis. For example, more recently, the first animal MRI and near-infrared fluorescence studies were conducted in AD models (37), even though the main focus was general brain activity. Generally, systemic (e.g., oral and intraperitoneal) exposure routes resemble the human situation closer. However, it is very difficult to give an exact indication of the amount of metal-intake (especially for oral regimes) and there are many substantial species differences in metal bioavailability between humans and rodents. Therefore, injections of predefined metal solutions directly into the brain allow a better control over brain concentrations and can target specific brain regions. Both injections of metal into the ventricle (i.c.v.) or into the hippocampus have been conducted successfully, combined with controls using vehicle injections. This regime is less variable, since systemic exposure routes have large variations between animals due to peripheral metabolism such as renal clearance, liver metabolism, and accumulation of toxins in adipose tissue or bone. In this paper, we have focused on the most common in vivo experimental models to study metal involvement in neurodegeneration typical of AD.
3.1 Aluminum
3.1.1 Aluminum Toxicity
Al represents about 8% of the Earth’s crust and is therefore the third most abundant element on Earth. Some researchers assert that in the “Aluminum Age” (38) in which we are living nowadays, we are subjected to continual chronic exposure from early embryonic development (39). An individual can be in contact with this metal from many environmental sources, through therapeutic treatments (e.g., antacid ingestion and vaccines) or through the use of certain cosmetic products. Moreover, Al is contained in many oven cooked foods, in certain other foods and beverages, in dust, and also in contaminants in potable tap water (40). In spite of its environmental abundance, no evidence exists to show a definite biological function for Al. On the contrary, it has been suggested that its chemical properties make it neutral or even incompatible with major physiological processes (41). Nevertheless, Al is not considered toxic in the same way as heavy metals, even if evidence of its toxicity when consumed in abundance does exist. Al toxicity, either in experimental animal models or in many different human clinical conditions, was the fulcrum of numerous scientific researches over the last century (42, 43). In 1886, Al was defined a neurotoxic metal for humans for the first time and the first research, which considered a relation between Al and certain alterations of the CNS, was published in 1937. From this moment, a wide ranging research has been carried out about the probable role of Al in dialysis syndrome (44), in cerebral functionality damages in some working categories (e.g., welders and foundry workers) (45), in amyotrophic lateral sclerosis (46), in Parkinson’s disease (47), and in AD (48, 49). Even if the role of this metal in the etiopathogenesis of these diseases is still in discussion, it has been clearly demonstrated that Al can interfere with a great number of metabolic and cellular pathways either in the CNS or in other bodily systems. Even after decades of studies on the biological role of Al, the exact mechanism by which it generates its neurotoxicity is still unclear.
3.1.2 Entry of Aluminum into the Body
To face the abundance and toxicity of Al, living organisms have developed active and passive mechanisms to remove this metal. In humans, these mechanisms include: (1) the reduction of Al absorption mediated principally by the liver, and also by particular human intestinal formation, (2) the excretion of Al through the kidney and, in smaller quantities, through the bile, (3) the reduction of plasmatic levels of Al by its sequestration in bones and by its bond to transferrin (Tf), the most important plasmatic protein for metal transport in the blood (40). Even given its ubiquitous presence, only 0.06–0.1% of ingested Al is absorbed into the gastrointestinal (GI) tract (50). This limited uptake of Al can be explained by considering the capability of certain dietary components, such as citrate and fluoride, to form complexes with Al (51) and also due to the competition of Al with Ca, Mg, and Si for absorption (52). Finally, vitamin D and parathyroid hormone also seem to interfere with Al absorption (53). The factor which above all influences Al absorption is pH. Many epidemiological studies and some studies about the complex chemistry of Al have demonstrated that at pH values close to 7.9, Al tends to form aggregates, which are more difficult to absorb, and can reduce its toxicity (54, 55). For this reason, if it is true that drinking tap water is the principal Al source for humans, its absorption probably occurs mainly through the skin, nasal and pulmonary surfaces (for example taking a shower or a bath) rather than through GI tract surfaces that are more acid and could theoretically allow large absorption of Al. It should be noted, however, that this has never been verified (40). Nevertheless, low Al absorption levels, as well as small amounts that reach the blood, could – over sufficiently long periods – cause significant accumulations and alterations in certain target organs such as bones, the erythropoietic system, and the CNS (56). This is especially so if Al cannot be removed because of alterations to kidney function. This damage can be explained if we consider that Al is a very chemically active element. In biological systems, it can form more stable complexes than those formed by other elements (such as Ca and Mg) and so it can substitute these elements when constituting certain biological molecules (e.g., proteins, ATP, GTP, nucleic acids, phosphates) and change their functions (57, 58). The alteration of these molecules can in its turn upset the balance of certain human systems and apparatus, particularly those with major accumulations of Al (59).
3.1.3 Aluminum and the Central Nervous System
The neuronal microenvironment and particularly constant chemical–physical parameters in the cerebral interstitial fluid are necessary to optimize neuronal functionality (60). The maintenance of these constant parameters over long periods is due to both the kidney filtering blood and the isolation performed by the blood-brain barrier (BBB). The maintenance of these parameters over short periods, however, is due to an integration between BBB action and glial cell activity. In the CNS of healthy individuals, lower Al levels are found than in other tissues or organs (61). This seems to be due both to its partial exclusion from the brain because of BBB action and to an active mechanism that allows Al efflux from the CNS, probably as Al-citrate (62). In his/her life, an individual is subject to chronic exposure to Al. This prolonged exposure can exert pressure on the maintenance of the physical–chemical equilibrium of the interstitial cerebral fluid (63). Al, in fact, can modify BBB permeability influencing molecules and ions flux towards and from the CNS (64). However, Al uptake in the CNS is not dependent on BBB permeability alterations (65, 66). Modifying the short-term regulation of cerebral interstitial fluid equilibrium, Al can dilute transduction molecule concentrations and by favoring large neuronal intakes of neurotoxins, reduce neuronal vitality. Three probable mechanisms by which Al enters the CNS from blood circulation have been proposed: (1) bypassing the BBB, (2) through the nasal-olfactory pathway (Al inhaled through the nose goes directly into CNS through the olfactory bulb), and (3) from cerebrospinal fluid (CSF) (61, 67). The most rapid Al intake in the CNS is certainly through the BBB, because many carriers with high affinity for this metal have been identified in the BBB. The best mechanism is Tf-mediated Al transport (65). Florence and colleagues showed an increase in transferrin receptor (TfR) concentrations in rat brains subjected to chronic oral administration of Al-citrate (Table 1) (68). Another equally important system is the monocarbossilic acid carrier (MCT), a proton cotransporter located on either side of the BBB (69). The MCT-mediated transport of Al into the CNS seems also to be closely dependent on the presence of free-citrate molecules, because of the use of citrates as a vehicle. The maintenance of Al cerebral homeostasis is either by its expulsion out of the CNS (70), or by its uptake by neurons and glial cells that compete to internalize Al (71, 72). Moreover, neurons are long life cells, so they can accumulate Al insight themselves (48). In maintaining Al homeostasis, Al uptake by nervous cells is also very important. Even if the internalization of Al is still not completely described, it probably involves different mechanisms such as endocytosis, facilitated diffusion, and active transport that determine the region-specific distribution of Al in the CNS (48). It is still very difficult to establish the real distribution of Al in the CNS because the methods presently used have a lot of analytical problems due to the complexity of Al chemistry. In healthy subjects, Al concentrations vary between 50 and 196 μg/g bodyweight depending on the specific cerebral region. It has been found in many cerebral areas (frontal, temporal, occipital, parietal and somatosensory cortex, hypothalamus, thalamus, hippocampus, and some cerebellar areas). The areas with the largest concentrations are certainly the temporal cortex and hippocampus, which are also those most involved in AD (41). An in vivo study by Fattoretti and colleagues reported an increase in Al3+ and other metals in the prosencephalon, mesencephalon, and pons-medulla of rats treated for 6 months with oral AlCl3 (Table 1) (73). All Al that bypasses the BBB to the CNS is divided in dynamic equilibrium in the extracellular environment, intracellular environment, and membranes. In the blood, Al binds to proteins (like Tf) and to other ligands with a low molecular weight such as small peptides, nucleotides, nucleic acids, citrate, phosphate, and silicic acid. In the interstitial cerebral fluid, Al also binds to certain neurotransmitters, such as GABA and glutamate. Al is associated to BBB surfaces and to plasmatic membranes of glial cells and neurons. Al may be bound by phosphate groups in the hydrophilic heads of membrane phospholipids, which in their turn act as aggregation sites for Al. At an intracellular level, Al may bind ATP, even if most is confined to the endoplasmic reticulum and Golgi regions near the nucleus. Some studies claim that Al is also linked to nuclear chromatin. Between extracellular and intracellular environments, there are continuous exchanges of Al that could influence the physiology of the CNS.
3.1.4 The Relation Between Al and Nitroxidergic System: A Possible Toxicity Mechanism
Al is considered a neurotoxic metal (74, 75) and therefore could be an etiologic factor in the pathogenesis of various nervous system diseases such as AD (48, 49). Different mechanisms are understood by which Al manifests its toxicity in nonnervous organs (free radical deposition and damage of the lysosomal system and cytoskeleton) (76). The molecular mechanisms by which Al is toxic, however, are not completely understood. Some research suggest that Al interferes with glutamatergic neurotransmission (77, 78), thereby damaging the glutamate (Glu) -nitric oxide (NO)- monophosphate cyclic guanosine (cGMP) pathway in vivo (79). In a 1999 study, oral chronic aluminum sulfate administration (3–5 weeks) increased extracellular cGMP by 240%, induced by nitric oxide-generating agents in rat brains (Table 1) (80). In this pathway, the activation of ionotropic glutamate receptors (especially NMDA) increases intracellular Ca2+, which, after binding with calmodulin, activates neuronal nitric oxide synthase (nNOS). NO is an atypical neurotransmitter because it does not accumulate in the synaptic vesicles, but is synthesized on request. In the CNS, NO is produced by nNOS using l-arginine as a substrate, and other synthesis factors (e.g., calmodulin, calcium, oxygen, NADPH, heme, and flavin). The neurons that produce NO are called nitroxidergic neurons. These represent about 1% of all neurons of the somatosensory cortex and are scattered among other neurons, which also act as interneurons (81). Once synthesized, NO spreads out of the presynaptic neuron that produced it, towards the postsynaptic neuron where it activates the soluble form of enzyme guanylate cyclases. This in turn increases cGMP concentrations (82). NO is a volumetric neurotransmitter, formed in one neuron; it can spread to nearby neurons and can activate their guanylate cyclases, which in their turn activate the Glu-NO-cGMP pathway in these neurons (79). This pathway is very important both under physiological and pathological conditions. Physiologically, it is fundamental because of its ability to modulate intercellular communication and important neurological processes, such as long-term potentiation, the basis of some forms of learning and memory (83, 84). Under pathological conditions, excessive activation of this pathway seems to be the basis for glutamatergic toxicity that is involved in the etiopathogenesis of many neurodegenerative diseases (80). A marked increase in Glu levels was observed in the hippocampus of albino rats treated for 35 days with aluminum sulfate (Table 1) (85). Neuronal NO was copurified with the enzyme NADPH-diaphorase (86–88). The role of Al in compromising the Glu-NO-cGMP pathway may be due to its ability to bypass the BBB and to form stable complexes with ATP, the principal transductional molecule for extracellular signals (89) and in the CNS, because it optimizes certain neurotransmitter activation (Glu, GABA, and acetylcholine) (90–92). Most ATP secreted in the interstitial cerebral fluid is combined with Mg, while only a little is free (93). When Al passes the BBB and penetrates into the CNS, it combines with ATP (both in cytosol and in interstitial cerebral fluid) more stably than with other metals (including Mg), thus exerting latent toxicity. If the Al–ATP complex replaces the Mg–ATP complex, it can potentiate glutamatergic neurotransmission (94). When, during glutamatergic neurotransmission, Glu and ATP are contemporarily released into the synaptic space, they act on their postsynaptic receptors to induce Ca2+ ion movements into nerve cells. In the presence of Al–ATP (rather than Mg–ATP), Ca channels remain open for too long and intracellular Ca2+ levels become too high. The consequent alteration in Ca2+ homeostasis compromises neuronal energy levels, causing neurotoxicity and neuronal death (95), in particular of nitroxidergic neurons. Therefore, some alterations in the Glu-NO-cGMP pathway can reduce nitroxidergic neurons and neuronal NO in certain cortical areas. These results were observed in AD patients’ brains (96, 97). The same decrease of nitroxidergic transmission has also been observed in rat brains treated with chronic oral aluminum sulfate. Recent studies showed that in these brains, the number of nNOS-expressing neurons decreased proportionally to the duration of Al treatment (Table 1) (98, 99). This further strengthens the hypothesis that Al in drinking water can be an etiological factor in AD (100).
3.1.5 Aluminum and Oxidative Stress
Al biochemistry is very particular because, even if Al is not a redox metal, it has clear pro-oxidant activity (101). This metal can promote the oxidation of biological molecules in both in vivo and in vitro experimental models. Its ability to favor Fe-dependent (102) and Fe-independent (103, 104) lipid peroxidation, to induce Fe-independent NADH oxidation (105) and to form hydroxilic radicals (106) have all been demonstrated. Numerous possible mechanisms to explain how Al could have pro-oxidant effects have been proposed. All seem to have in common the formation of a very reactive Al species, i.e. the radical ion semi-reduced superoxide Al, which produces, due to its interaction with Fe, free radicals of oxygen both under pathological and physiological conditions (101). Al probably acts by stabling ferrous ions (Fe2+), which are potent promoters of oxidative species generation as they catalyze Fenton’s reaction, which forms ferric ions (Fe3+) and OH−. Moreover, Al is an activator of superoxide dismutase (SOD), the enzyme, which catalyzes hydrogen peroxide (H2O2) synthesis from superoxide ion, and an inhibitor of catalase, the enzyme which transforms H2O2 in less reactive species. Al can therefore increase H2O2 levels that, in turn, increase oxidative species production able to damage proteins, DNA, and membrane lipids causing oxidative stress inside cells. Numerous in vivo studies have confirmed Al’s ability to induce oxidative stress. Yuan and colleagues showed increased expression and activity of HO-1 (a stress protein) in the hippocampus of elderly mice after an i.c.v. injection of aluminum chloride (Table 1) (107). Rodella and colleagues demonstrated a clearly reduced neuronal expression of GRP78 (another stress protein) after chronic exposure over 12 months to aluminum sulfate in mice (Table 1) (108).
3.1.6 Aluminum and β-Amyloid
Al, besides being a constituent of senile plaques, promotes Aβ aggregation (increasing it 100–1,000 times) and can increase its toxicity (109). This was confirmed by a recent study showing a higher amount of Aβ fragments in APP transgenic mice brains after Al administration, compared to nontreated transgenic mice (Table 1) (110). Another characteristic of Al is its ability to increase posttransductional modifications of APP and, therefore, to accelerate the production of Aβ after APP proteolysis. Al aids serine proteases (hampering its inhibitors) and so facilitates Aβ production and its accumulation and aggregation in senile plaques (111). Aβ is a metalloprotein able to bind ions of certain transition metals such as Zn, Cu, and Fe. This explains why high levels of these metals have been found in senile plaques (20). The bond between Aβ and the redox ions Cu and Fe overproduces H2O2, which causes oxidative damage and neuronal dysfunction by reducing them. Some researchers conversely believe that Aβ could be nontoxic itself, but toxic for neurons because of its ability to increase H2O2-mediated oxidative damage. The formation of plaques could compensate oxidative stress because, if Aβ binds metal ions in excess, it can chelate them from the action of H2O2 (112). Despite these contrasting results, most experimental evidence indicates that Al accumulation in brain tissue may have neuropathological relevance. In view of the great social impact of AD in which Al seems to be implicated, continued research is clearly justified (113, 114) (Table 1).
Table 1
Animal models of aluminum-induced dementia
Reference | Experimental model | Exposure protocol | Effects |
---|---|---|---|
(73) | Male Wistar rats (22 months old) | Chronic (6 months) Al (AlCl3 × 6H2O) administration (2 g/L) in drinking water | Increased contents of Al3+, Cu2+, Zn2+, and Mn2+ prosencephalon, mesencephalon, and pons-medulla; |
No change in the cerebellum except a Cu2+ decrease | |||
Increased area occupied by the mossy fibers in the CA3 field (+32%) | |||
(80) | Male Wistar rats | Chronic administration (3–5 weeks) of aluminum in drinking water (2.5% aluminum sulfate) | Increase in extracellular cGMP induced by nitric oxide generating agents (240%) |
Decreased cerebellar content of calmodulin (34%) and nitric oxide synthase (15%) | |||
Decreased basal activity of soluble guanylate cyclase (66%) | |||
Decreased basal cGMP in the cerebellar extracellular space (50%) | |||
(108) | C57BL6 mice (5 weeks old) | Chronic exposure over 12 months to aluminum sulfate (2.5%) in drinking water | Deposition of Aß similar to that seen in congophilic amyloid angiopathy (CAA) in humans |
Reduction in neuronal expression of GRP78 similar to what has previously been observed in AD | |||
(98) | Male Sprague–Dawley rats | Aluminum sulfate 2.5% in tap drinking water (corresponding to 0.2% Al) for 0.5, 1, 2, 3, 6, and 12 months | Reduced NADPH-d and nNOS positive neurons in the cerebral cortex time dependently, with the greatest effect appearing after 3 months |
Decrease in NADPH-d/NPY double-stained neurons with an apparent increase in NPY single-stained neurons between 1 and 3 months of exposition (these then decreased after 6–12 months) | |||
(85) | Male albino rats | Aluminum sulfate (Al2(SO4)3 ·18H2O) by gavage (21.2 mg/kg b.w.) for 35 days | Marked increase in glutamate and glutamine levels; significative decrease in GABA levels; spongioform changes in the neurons of hippocampus |
Nuclear deformity, and neurofibrillary degeneration, similar to NFT in AD | |||
(113) | Wistar rats | Al lactate (10 mg/kg of body weight/day) for 4 weeks through I.P. injections | Lower levels of acetylcholine |
Significant decrease in high-affinity choline uptake in the hippocampus; neurobehavioral deficits in terms of decreased active (52%) and passive (73.30%) avoidance tests | |||
(110) | Tg2576 mice (5 months of age) | Diet supplemented with Al lactate (1 mg/g of diet) for 120 days | Impaired acquisition and retention of the water maze task |
Higher amounts of Aß fragments in brain | |||
Increase in the total number of proliferating cells in the dentate gyrus of hippocampus | |||
(114) | Female Tg2576 mice and wild-type littermates | Diet supplemented with 1 mg Al/g for 6 months | In hippocampus, Cu concentrations decreased in nontreated mice, while Zn levels were higher in Al-treated mice (at 11 months) |
Not important metal changes | |||
(107) | Old mice | 2 µL of 0.25% aluminum chloride solution (i.c.v. injections once a day for 5 days) | Increased expression of HO-1 protein, and elevation of both HO activity and iron level in hippocampus |
(68) | Male Wistar rats | Chronic oral administration (6 months) of Al citrate | Increased brain Al levels by 30-fold; |
Al tissue content significantly greater when the Al citrate was administered in an iron-free diet (distribution of Al in brain similar to that AD) | |||
Higher concentrations of transferrin receptors | |||
At the cellular level an extensive cytoplasmic vacuolation in astrocytes (especially) and neurons |
3.2 Iron
3.2.1 Iron Use in the Body and Brain
Fe is essential for life. Adequate Fe is important, because Fe deficiency causes anemia, which can lead to fatigue, decreased exercise tolerance, and general inanition. Thus, evolution has created reserves to balance periods of relative loss from such things as hemorrhage or bleeding. Intake of adequate bioavailable Fe, usually present in meat, is necessary to prevent anemia. Fe is found in the active centers of many enzymes and oxygen carrier proteins. It is a central element of the heme molecule, a critical part of hemoglobin essential for oxygen transport. Heme is also part of many essential enzymes, such as the cytochrome series, and Fe is found in cytochrome oxidase and in the iron–sulfur complexes of the oxidative chain important for producing ATP (115). Some enzymes involved in DNA synthesis, and succinate dehydrogenase of the tricarboxylic acid cycle, are also Fe-dependent (116). All these metabolic processes take place in the brain at a relatively high level. Indeed, the brain, which only takes up 2% of the total body weight, accounts for 25% of oxygen consumption, making the brain the highest oxygen-consuming organ in the body. Consequently, oxygen transportation and its associated metabolic activities are relatively high in the brain and create a high demand for Fe transportation to and use within the brain. Therefore, Fe is important to maintain many specific neurobiological processes. Additional demands of Fe in the brain come from myelogenesis and myelin maintenance. Oligodendrocytes, the cells responsible for myelin production and maintenance, are Fe-rich compared to other brain cells (117). The synthesis of neural transmitters is also Fe-dependent. Fe is needed as a cofactor to produce dopamine, norepinephrine, and serotonin, which play a significant role in certain chronic neurological disorders (118). There is also intense Fe staining in brain areas that receive GABA-ergic innervation (119) suggesting a possible relation between Fe and this inhibitory neurotransmitter.
3.2.2 Intra- and Extracellular Iron Transport
The dietary Fe pathway to the brain begins in the intestines where Fe3+ (ferric iron) is reduced by duodenal cytochrome-b to Fe2+ (ferrous iron). In this reduced form, the divalent metal transporter can carry Fe across the duodenal epithelium into the blood. In the blood, Fe2+ is oxidized to Fe3+ by ceruloplasmin to be coupled with Tf, which has two Fe-binding domains that bind the ferric form with very high affinity (120). Altered location of the Fe-transport protein Tf in senile plaques, rather than its regular location in the cytosol of nervous cells, may suggest that Tf becomes trapped within plaques when it attempts to transport Fe between cells (121). Studies examining Tf to Fe ratios in brain show that this ratio decreases in AD brains (122). This suggests a decrease in Fe mobility in AD patients consistent with the idea that the decrease in Tf in AD brains could be associated with a deficit in intracellular brain Fe mobilization or with a lower efflux of Fe from the brain. Such a decrease would increase Fe “labile pools” and would contribute to the increased susceptibility of the AD brain to Fe-induced oxidative damage (123). Furthermore, increased Tf subtype C2 frequency was reported in patients with AD (124). The presence of the C2 variant plus a hemochromatosis mutation increased the risk of AD fivefold, which was increased even more in the presence of APOE-ε4 allele (125). Fe homeostasis maintained in the brain is similar to systemic Fe homeostasis, although Fe circulating in the blood outside the CNS cannot directly cross the BBB. There are several pathways that can transfer Fe across the BBB. The first and probably most common pathway for Fe uptake in the brain is tightly controlled by the TfR expressed in neurons, in the endothelial cells of cerebral blood vessels, and in choroid plexus cells (126) that bind Fe circulating when bound to transferrin. The TfR-bound complex then enters the brain by endocytosis. In AD, significant decreases in TfR density were noted in the hippocampus and temporal and occipital cortices, but TfR density was unchanged in microvasculature, parietal and frontal cortices, and cerebellum (127). These data, even if difficult to understand, suggest that TfRs decrease in areas associated with memory loss (128). Several other transporter systems may also deliver Fe across the BBB, such as the divalent metal transporter and the lactoferrin receptor (35). A critical component of cellular Fe transport is the mechanism by which Fe is released into the cytoplasm. Removal of Fe from Tf within the endosome requires lower pH within the endosome itself. Decreased pH causes a lower affinity of Tf for Fe so that Fe dissociates from the carrier. Once Fe has been released into the cytosol from endosomes, it is either incorporated into Fe-requiring enzymes or exists at low concentrations (labile Fe pool).
3.2.3 Intracellular Iron Homeostasis
Labile Fe is potentially toxic and therefore, as a protection mechanism, cells have evolved an extensive and elegant control mechanism to regulate intracellular Fe bioavailability. Fe that is not immediately used in the cell is stored in ferritin. Ferritin is the most common Fe-storage protein in the brain and can store up to 4,500 Fe3+ atoms as an inorganic complex (129). Serum ferritin provides one measure of Fe storage, and a low ferritin blood level is a reliable indicator of Fe deficiency. It consists of two types of subunits, the heavy and light chains that work in a complementary way to store intracellular Fe. Heavy ferritin (21 kDa) efficiently sequestrates Fe and is found in organs with high Fe use and little Fe storage, while light ferritin (19 kDa) is associated with Fe storage (130). Studies by Connor and colleagues (131) found variations in ferritin levels. In elderly subjects, an increase in heavy and light ferritin coincides with an age-related increase in Fe. In AD, however, there is no concomitant increase in ferritin, despite Fe levels increase. In addition, ferritin isolated from the brains of AD patients has a higher Fe content than ferritin isolated from control brains (132). Together, these data suggest that Fe levels are increased in the brains of AD patients relative to ferritin levels. The rise in Fe levels without a simultaneous change in ferritin provides a source of labile Fe that generates free radicals. Finally, after the brain uses the Fe it has stored, it must leave the cells and the Cu-associated protein ceruloplasmin may facilitate cellular release of Fe (133). The importance of ceruloplasmin in brain Fe metabolism is highlighted by the extreme accumulations of Fe in patients with hereditary aceruloplasminemia (134). Fe leaves the body via bleeding or through the shedding of skin and other cells (135). Recently, it has become clear that the regulation/management of Fe at a cellular level, although primarily by Tf, TfR, and ferritin, is also controlled by lactotransferrin receptors, melanotransferrin, ceruloplasmin, and divalent cation transporters. So, disruption in these proteins can contribute to the altered brain Fe metabolism found in neurodegenerative disorders (20). Control at a posttranscriptional level of these proteins and therefore overall regulation of cellular Fe metabolism involves the action of two Fe regulatory proteins, IRP-1 and IRP-2. Iron responsive elements (IREs) found on certain mRNAs are targets for high-affinity interactions with the cytoplasmic protein IRP. The interaction of IRPs with IREs controls the translation or stability of mRNA (136). When intracellular Fe levels decrease, the affinity of IRP for IRE increases. IRPs bind to the IREs on 59UTR ferritin mRNA, preventing initiation of ferritin translation and decreasing cellular Fe storage capacity (137). At the same time, other IRPs can bind to IREs in the 39UTR of TfR mRNA, inhibiting degradation of TfR mRNAs and increasing TfR levels. Conversely, high intracellular Fe levels promote dissociation of IRPs from IREs on ferritin and TfR mRNA causing increased ferritin mRNA translation and decreased stability of the TfR mRNA (138). IRP-1, but not IRP-2, is rapidly activated by extracellular H2O2, establishing a regulatory connection between Fe metabolism control and oxidative stress response. IRPs are therefore critical to maintain cellular Fe homeostasis that could become dysfunctional in neurodegenerative disorders. Studies by Yamanaka and colleagues (139) showed that Al may stabilize IRP2, increasing its ability to bind to IREs. Further evidence for a disruption in the IRPs in AD comes from the immunocytochemical studies by Smith and colleagues who showed a selective association of IRP2, but not IRP1, with the pathological hallmarks of AD localizing to neurofibrillary tangles (NFT), senile plaques (SP), and neuropil threads (i.e., the neurofibrillary lesions) (140).
3.2.4 Iron and Oxidative Stress
The physiological, biochemical, and anatomical aspects of Fe homeostasis must be considered to understand the role of this metal in chronic neurological disorders. Although Fe’s importance for normal neurological activity is clear, too high Fe concentration may have devastating effects. Fe is one of the most essential transition metals involved in the formation of oxygen-free radicals, owing to its interaction with H2O2 through Fenton chemistry. During the reduction of molecular oxygen, mitochondria produce superoxide (O2 −). Enzymatic dismutation by SOD in turn yields H2O2. Although O2 − and H2O2 by themselves are relatively nontoxic, H2O2, which is freely permeable in tissues, could produce highly toxic hydroxyl radical (OH−) through a metal Fe-catalyzed Fenton reaction. This reaction is referred to as the superoxide-driven Fenton reaction. Superoxide may also produce H2O2 nonenzymatically. In its normal metabolic state, superoxide favors the oxidation of Fe2+ to Fe3+. However, if the intracellular concentration of superoxide is high, the reaction helps reduce Fe3+ to Fe2+ and elaboration of hydroxyl radicals. In light of the high levels of oxygen consumption in the CNS, high levels of reactive oxygen species (ROS) are expected, as well as antioxidant defenses commensurate with free radical production. Under normal situations, oxidative balance is maintained and free radicals are detoxified. In disease, various modifications of macromolecules such as sugars, lipids, proteins, and nucleic acids, can lead to critical failure of biological functions and ultimately cell death (141, 142). It is now well established that neurodegenerative diseases are associated with oxidative imbalance and its consequences. Abnormally high levels of Fe have been found in a number of neurodegenerative disorders. Importantly, increased total Fe does not necessarily imply increased oxidative stress if there are concomitant increases in proteins that store Fe in redox inert forms. For example, ferritin (which may be upregulated in AD) contains a core of insoluble, unreactive ferrihydrate. Microglia are the major sites of ferritin bound Fe and are thought to be partly responsible for oxidative damage in neurodegenerative disorders. Interesting, oxidant damage is intimately involved with aging diseases, such as atherosclerosis, autoimmunity diseases, diabetes, fibrosis, inflammation, Parkinson’s, and AD.
3.2.5 Iron Overaccumulation in Brain Regions Susceptible to AD
It has become apparent that Fe progressively accumulates in the affected brain areas of neurodegenerative disorders (143, 144). Overaccumulation of Fe in AD has been found within specific brain regions displaying selective vulnerability to neurodegeneration such as the hippocampus, cerebral cortex, and basal nucleus of Meynert (20, 145). Using an in situ Fe detection method, Sayre and colleagues (146) found redox-active Fe associated with both NFT and senile plaques. The association of Fe with NFT may be in part related to Fe binding to their primary protein constituent, tau. These results add to earlier studies reporting increased levels of Fe, Tf, and ferritin in AD. Further studies have suggested that accumulated Fe supports the AD pathology as a possible source of oxidative stress, demonstrating that neurons in AD brains have high oxidative loads (147, 148). Intrahippocampal unilateral infusion of a ferrous citrate solution in rats causes an increase in lipid peroxidation levels that extensively damage neurons of hippocampal regions (Table 2) (149). Moreover, de Lima and colleagues showed extensive oxidative damage (increased superoxide production and SOD activity) in rats’ brains treated for a few days with oral Fe2+ (Table 2) (150). Postmortem analysis of Alzheimer patients’ brains has revealed the activation of two enzymatic indicators of cellular oxidative stress: heme oxygenase (HO-1) (151) and NADPH oxidase (152). HO-1 was greatly increased in neurons and astrocytes of the hippocampus and cerebral cortex of Alzheimer subjects, colocalizing with senile plaques and NFT (153). HO-1 catalyzes the conversion of heme to Fe and biliverdin, which in turn, is reduced to bilirubin, an antioxidant. Since HO-1 is induced in proportion to the level of heme, HO-1 induction would suggest abnormal turnover of heme in AD. This may be connected to mitochondrial abnormalities in AD, which contain numerous heme proteins. Fe accumulation in AD has been shown to be particularly abundant in brain regions vulnerable to AD and at the microscopic level, it accumulates in senile plaques in AD (20). Increases in Fe accumulation and oxidative stress in AD brains are related to changes in the concentration of soluble and deposited Aβ protein. At a biochemical level, Aβ is defined as a redox-active metalloprotein that is precipitated by interactions with neocortical metal ions, especially Zn, Cu, and Fe resulting in its auto-aggregation and oligomerization (154, 155). Several studies have shown that oxidative stress increases Aβ production (156). On the other hand, Aβ itself may be able to generate ROS, driving a potential positive feedback loop whereby increased generation of ROS increases Aβ and vice versa. Three histidine and one tyrosine residue at the hydrophilic N-terminus of the peptide are crucial to bind metal to the peptide (157). Regarding Fe interaction, Fe3+ influences the formation of Aβ1–42 fibrils (158). The interaction of redox-active Fe3+ with Aβ fibrils causes their chemical reduction and generation of reactive oxygen species, causing lipid membrane peroxidation, DNA breakdown, and protein oxidation (159). In vitro, Fe is needed for Aβ toxicity, since Fe deletion from the culture medium or the inclusion of adequate Fe chelators reduces or blocks toxicity, while overloading cells with Fe increases toxicity (160). Hence, abnormal Fe deposition, such as in AD plaques, may interact with Aβ to mediate free-radical-induced neurotoxicity and account in part, for the widespread oxidative damage in AD brains, accelerating the pathological process (161). Unexpectedly, the redox potential of Fe is significantly attenuated by Aβ, suggesting a neuroprotective chelating role for Aβ in disease pathogenesis (162). NFT are another hallmark lesion of AD and, interestingly, are another site of Fe accumulation. The presence of redox metals in NFT has been shown to induce oxidative stress via H2O2 (146). Fe3+ has the ability (similar to Al3+) to bind with hyperphosphorylated tau and to induce its aggregation in vitro, leading to the formation of NFTs in AD. Although NFTs possibly act as a redox center, neurons lacking NFTs also contain oxidative modifications (163), suggesting that oxidative stress precedes NFT formation. Among the macromolecules modified by ROS, RNA may be an early target of oxidative damage in AD brains (164). Using 8-hydroxyguanosine as a marker of nucleic acid oxidation, oxidative damage can be found within the neuronal perikaryal cytoplasm. It is therefore possible that transition metals such as Fe play a pivotal role in the oxidation of RNA. Studies show that RNA oxidization is increased in AD brain (165) and subsequent protein translation is impaired (166) (Table 2).
Table 2
Animal models of Iron induced dementia
Reference | Experimental model | Exposure protocol | Effects |
---|---|---|---|
(149) | Male Wistar rats | Intra hippocampal unilateral infusion of 1 µL Kreb’s Ringer solution containing ferrous citrate (iron: 3.4 mmol/L) at a rate of 0.2 µL/min | Increase in lipid peroxidation levels; extensive damage in neurons from the CA1 and CA3 regions of the Fe2+-treated animals |
(150) | Male Wistar rats | 10.0 mg/kg of oral Fe2+at postnatal days 5–7, 12–14, 19–21, or 30–32 | Impairments in long-term retention of object recognition memory |
Oxidative damage in the brain (increased superoxide production in submitochondrial particles) | |||
Increased superoxide dismutase activity in brain |
3.3 Lead
3.3.1 General Aspects
Pb is a neurotoxin that continues to be considered a global environmental health hazard. In particular, the ability of Pb to interact in a flexible coordination with protein oxygen and sulfur atoms and form stable complexes with them increases its affinity for proteins when compared to other metals (167). Pb is a heavy metal with no known biological function in humans. Susceptibility to Pb toxicity is influenced by several factors such as environmental exposure, age, and nutritional status. Human exposure to Pb occurs via food, water, air, and soil. It has been used for various applications in glass, pigments, makeup, water transport, wine, cooking, and more recently in antiknock fuel additives, electronic components, and batteries. People can also be exposed to Pb contamination from industrial sources, e.g., smelters and workers of Pb manufacturing industries (168). Thus, Pb is still a significant public health concern. Pb toxic effects depend on both the duration of exposure and the magnitude of the dose. Pb has well-characterized effects on every organ system, including cardiovascular (169), renal (170), immune (171), and reproductive (172) systems, as well as on bones and teeth (173). It has also been identified as a probable human carcinogen (174). However, the nervous system is especially sensitive to Pb effects. Additionally, it has been shown that Pb is stored in bone and released over time, especially during periods of bone demineralization such as pregnancy, lactation, and postmenopause. High Pb exposure in pregnant women can cause a low infant birth weight or prematurity. In addition, when the Pb level in maternal blood increases, Pb levels in breast milk also increase with additional risks to the neonate (175). Children are particularly sensitive to the deleterious effects of Pb. They can absorb 30–75% of Pb ingested (176), while adults absorb about 11% of Pb. In addition, pre- and perinatal exposure to Pb causes higher brain metal accumulation than later postnatal exposure due to an underdeveloped BBB in early life. The consequences of high blood Pb levels in children may be irreversible, including learning disabilities (177), behavioral problems, and mental retardation. At very high blood concentrations, Pb can cause convulsions, coma, and even death. Nutritional status is another significant risk factor for Pb intoxication. Fe, Zn, and Ca deficiencies increase the retention of ingested Pb, which can also increase Pb GI absorption (178), and affect Pb neurotoxicity susceptibility (179).
3.3.2 Lead Neurotoxicity and Its Possible Link with Alzheimer’s Disease
The mechanisms underlying Pb neurotoxicity are still a matter of debate. So far, the effects of Pb on Ca fluxes and metabolism have been suggested as major mechanisms involved in Pb toxicity (180). Other potential mechanisms for Pb toxicity include its capacity to affect cell membrane biophysics, cause oxidative stress, and trigger certain transcription factors sensitive to oxidative status of the cell. Experimental studies have demonstrated that environmental factors (such as stress) can interact with Pb exposure. Bolin and colleagues found changes in the expression of copper/zinc SOD, manganese SOD, and reduced-form glutathion in rat brains after short-term or long-term lead acetate administration in drinking water (Table 3) (181). Tiffany-Castiglioni and colleagues showed that Pb binds to GRP78 during the Pb accumulation process in astroglial cells (182). As GRP78 is a stress protein, this binding may contribute to increased susceptibility of the brain to stress. Chaperone deficiency is implicated in a number of CNS diseases, including AD. The work published by Stewart and colleagues (183) revealed occupational exposure to Pb as a risk factor for AD. In this seminal work, the authors looked at the relation of Pb bone levels to the ApoE genotype. They concluded that the persistent CNS effects of Pb are more toxic in individuals with at least one APOE-ε4 allele (183). Momcilovic and colleagues found a tenfold increase of Pb in the white matter of AD patients (184). Moreover, Zawia and colleagues reported that Pb exposure in early life in both rodents and monkeys upregulates APP mRNA expression and increases the levels of its amyloidogenic cleavage product Aβ (Table 3) (185–187). These studies showed an association between developmental Pb exposure and amyloidogenesis during old age. They propose epigenetics as one of the potential mechanisms that mediate its involvement in AD etiopathogenesis and suggest that early Pb exposure may inhibit the methylation of CpG dinucleotides in the APP promoter, causing increased responsiveness of the APP gene later in life to transcriptional factors. This increased responsiveness could be the principal reason for upregulation of APP mRNAs in AD brains. In support of this, Wu and colleagues demonstrated decreased DNA methyltransferase activity and higher levels of oxidative damage to DNA in monkey brains treated with 1.5 mg kg−1 day−1 of lead acetate from birth until 400 days of age (Table 3) (188) (Table 3).
Table 3
Animal models of Lead-induced dementia
Reference | Experimental model | Exposure protocol | Effects |
---|---|---|---|
(188) | Female monkeys (Macaca fascicularis); the monkeys were terminated 23 years later. | 1.5 mg kg−1 day−1 of lead acetate from birth until 400 days of age via infant formula and vehicle after weaning | Elevated expression of AD-related genes (APP, BACE1 (beta-site APP cleaving enzyme 1)] and their transcriptional regulator (Sp1). |
Alterated levels, characteristics, and intracellular distribution of Abeta staining and amyloid plaques in the frontal association cortex. | |||
Decrease in DNA methyltransferase activity and higher levels of oxidative damage to DNA. | |||
(186) | Long–Evans males hooded rats | 200 ppm Pb (lead acetate) from day 1 after birth to day 20 through the drinking water or from18 to 20 months of age. | Formation of Abeta aggregates |
Developmental exposure to Pb did not alter the lifetime profiles of alpha-, beta-, and gamma-secretases in adult and aging animals. | |||
(186) | Long–Evans males hooded rats. | 200 ppm Pb (lead acetate) from day 1 after birth to day 20 through the drinking water or from 18 to 20 months of age | Transient induction of APP mRNA expression in neonates, but it exhibited a delayed overexpression 20 months after exposure to Pb had ceased. |
Rise in activity of the transcription factor Sp1 (a regulator of the APP gene). | |||
(181) | Timed-pregnant Long–Evans hooded rats. | 0.2% Lead acetate from postnatal day 1 through postnatal day 20 through the drinking water or from 18 to 20 months of age | 20 months after Pb exposure stable induction of cerebral 8-hydroxy-2′-deoxyguanosine (Oxo8dG) |
Not altered DNA repair enzyme 8-oxoguanine DNA glycosylase (Ogg1) activity | |||
Changes in copper/zinc-superoxide dismutase (SOD1), manganese-SOD (SOD2), and reduced-form glutathion (GSH). |
3.4 Manganese
3.4.1 General Aspects
Mn exists in a number of physical and chemical forms in the Earth’s crust and it can assume different oxidation states. In living tissue, Mn has been found as Mn2+, Mn3+, and possibly as Mn4+ (189). Mn tends to form very tight complexes with various substances (190). As a result, its free plasma and tissue concentrations tend to be extremely low (191). In adults, dietary Mn concentrations influence the amount of Mn absorbed from the GI tract and the amount eliminated in bile. When dietary Mn levels are high, adaptive changes reduce GI absorption, enhance liver metabolism, and increase its biliary excretion (192). Thus, despite large fluctuations in oral Mn intake, brain and other tissue Mn levels remain relatively constant, at least in healthy adults. Recent studies suggest that high levels of Mn in drinking water (>300 mg/L) are associated with reduced intellectual function in children (193). High exposure to airborne Mn has also been associated with severe neurotoxic effects (194). Mn is an essential metal found in a variety of biological tissues and is necessary for a variety of physiological processes including amino acid, lipid, protein, and carbohydrate metabolism (195). In the brain, Mn is an important cofactor for a variety of enzymes, including the antioxidant enzyme SOD (196), as well as enzymes involved in neurotransmitter synthesis and metabolism (197). In excessive quantities, however, it can cause damage to the CNS. Mn enters the brain from the blood either across the cerebral capillaries and/or the cerebrospinal fluid. At normal plasma concentrations, Mn appears to enter the CNS primarily across the capillary endothelium, whereas at high plasma concentrations, transport across the choroid plexus appears to predominate (198), consistent with observations on the rapid appearance and high levels of Mn in this organ (199). It would appear that facilitated diffusion (198), active transport (198), divalent metal transporter 1-mediated transport (200), ZIP8- and Tf-dependent transport mechanisms (201) are all involved in shuttling Mn across the BBB.
3.4.2 Manganese and Manganism
Since the last century, the neurotoxicity of Mn has been well known (202) as manganism, and has been described and characterized by extrapyramidal dysfunction and neuropsychiatric symptoms. This syndrome has been observed throughout the world in hundreds of cases among miners and industrial workers who had been exposed to high levels of Mn (203). Cases of overt Mn poisoning were also reported due to environmental exposure in drinking water in Japan (204), or after drinking water low in magnesium but with a diet high in Mn content (205). Another possible source of Mn accumulation and clinical toxicity has been identified in excessive Mn levels in total parenteral solution, given intravenously to patients with chronic GI illnesses (206). In addition, since Mn is excreted almost completely via the biliaric tract, individuals with chronic liver failure are at the risk of developing hepatic encephalopathy, probably due to Mn accumulation in the brain (207).
3.4.3 Neurotoxicity of Mn: Parkinsonian Disturbances
Since Mn elimination from the CNS requires a long time, neurotoxic effects may occur later in life, increasing the frequency of Parkinsonian disturbances in elderly individuals. The signs and symptoms from relatively high levels of exposure include postural instability, mood and psychiatric changes (i.e., depression, agitation, hallucinations) (208), and Parkinsonian symptoms such as bradykinesia, rigidity, tremor, gait disturbance, postural instability, and dystonia and/or ataxia (209). Cognitive deficits such as memory impairment, reduced learning capacity, decreased mental flexibility, cognitive slowing (209), and difficulty with visuomotor and visuospatial information processing (210) have also been reported. The adverse effects of Mn on the nervous system are probably due to the failure of protective enzymes capable of detoxifying critical amounts of Mn or to alter its oxidation potential. On the one hand, the basis of Mn neurotoxicity hinges on the ability of the bivalent form Mn2+ to oxidize to the trivalent form Mn3+, a powerful oxidizing species. On the other hand, Mn shows high affinity to neuromelanin-rich areas, such as the nigrostriatal tract (211). At this site, Mn favors the autoxidation of dopamine, generating toxic free radicals (212), which can cause substantial injury of the dopaminergic system. A multifactor hypothesis is more likely and could involve iron-induced oxidative stress and the direct interaction of Mn with dopaminergic terminal mitochondria, leading to selective mitochondrial dysfunction, subsequent excitotoxicity, and finally, neurodegeneration (202).
3.4.4 Manganese and Alzheimer’s Disease
Although Mn has no reported direct interaction with Aβ, recent reports have highlighted the impact of the loss of Mn superoxide dismutase (MnSOD) on AD in APP transgenic models. MnSOD is found exclusively in mitochondria, and heterozygotic genetic ablation of MnSOD leads to increased oxidative stress. Mice lacking MnSOD die within the first week of life, and develop a complex heterogeneous phenotype arising from mitochondrial dysfunction and oxidative stress. Treatment of these mice with catalytic antioxidants increases their lifespan and rescues the peripheral phenotypes, while uncovering the CNS pathology. MnSOD null mice exhibit striking increases in tau phosphorylation, which can be remedied with an SOD-mimetic compound, previously reported to be sufficient to prevent neuropathology (Table 4) (213). Crossing MnSOD± mice with Tg2576 APP transgenic mice leads to increased amyloid burden and tau hyperphosphorylation (Table 4) (213). In similar studies, the genetic combination of MnSOD± with the Tg19959 model of AD increased amyloid burden (Table 4) (214). However, a more recent report observed decreased plaque burden in the parenchyma, with increased vascular amyloid, gliosis, and exaggerated neurocognitive deficits as a consequence of MnSOD heterozygosity in J20 APP transgenic mice (Table 4) (215). Another report showed gliosis and the development of pathological changes in glial morphology after intraperitoneal injection of manganese chloride in rats (Table 4) (216). Taken together, these reports indicate that MnSOD-induced mitochondrial oxidative stress affects AD-like pathologies, but clearly the effects vary according to the AD mouse model used. This raises the fact that Mn deficiency might increase the risk for AD. Mn nutritional status and homeostasis is an underexplored area and warrants more attention (Table 4).
Table 4
Animal models of Manganese-induced dementia
Reference | Experimental model | Exposure protocol | Effects |
---|---|---|---|
(216) | Wistar rats | Manganese (II) chloride (50 mg/kg body weight, i.p.) once daily for 1 or 4 days | Increases in manganese levels of up to 232%, 523%, and 427% in the cerebral cortex, globus pallidus, and cerebellum; development of pathological changes in glial morphology identified as Alzheimer type II astrocytosis in both cortical and subcortical structures |
(213) | MnSOD null mice (a) and heterozygous MnSOD knockout mice combined with a well-characterized mouse model of AD (Tg2576) (b) | High and low doses of a catalytic antioxidant | (a) Striking elevations in the levels of tau phosphorylation (at Ser-396 and other phospho-epitopes of tau) in the low-dose antioxidant treated mice at AD-associated residues |
(b) Exacerbated amyloid burden and significantly reduced metal levels in brain | |||
Increased levels of Ser-396 phosphorylated tau | |||
(215) | Human amyloid precursor protein (hAPP) transgenic mice | Inactivation of one MnSOD allele (MnSOD(±)) reducing MnSOD activity to approximately 50% | Decreased amyloid plaques in the brain parenchyma |
Development of cerebrovascular amyloidosis, gliosis, and plaque-independent neuritic dystrophy | |||
(214) | Mice with a knockout of one allele of MnSOD crossed with Tg19959 mice (overexpression of a doubly mutated human APP) | Elevated oxidative stress and significantly increased brain Abeta levels and Abeta plaque burden |
3.5 Copper
3.5.1 Copper Metabolism
Cu is an essential component of countless enzymes and other proteins and it is critical for numerous vital reactions. Cu homeostasis is complex and the mechanisms of Cu trafficking, metabolism, and sequestration are currently being studied (217). A healthy human contains about 110 mg of Cu, with about 9 mg present in the brain (218, 219). There is a continual turnover of Cu, with most of it being recycled. Daily diets have around 0.6–1.6 mg of Cu (contained especially in meat), but only about half of this ingested metal is absorbed into the small intestine (220) and then transported into blood. Cu transport is dependent on its own oxidation state: only Cu reduced by specific metalloreductases, i.e. Cu(I), can be transported. About 90% of blood Cu in humans is covalently bound to ceruloplasmin, while the remaining 10% is free or loosely bound to albumin and other molecules. Excess of free Cu (free or noncovalently bound Cu) is toxic to cells because of its ability to promote uncontrolled forms of reactive species through Fenton chemistry (Cu is a transition metal). It is therefore essential that mechanisms exist to sequestrate free intracellular Cu. Metallothionein is a cellular Cu-binding protein, which provides Cu storage in the liver, giving significant protection against high free Cu levels. It is a regulatory mechanism, which balances Cu import and export to ensure proper nutrient levels while minimizing toxic effects (221). Several neurodegenerative diseases are characterized by altered Cu homeostasis, which directly or indirectly increases oxidative stress, an important factor in neuronal toxicity (222). In particular, growing evidence supports a central role for altered Cu metabolism at many steps in development and progression of AD (155).
< div class='tao-gold-member'>
Only gold members can continue reading. Log In or Register a > to continue
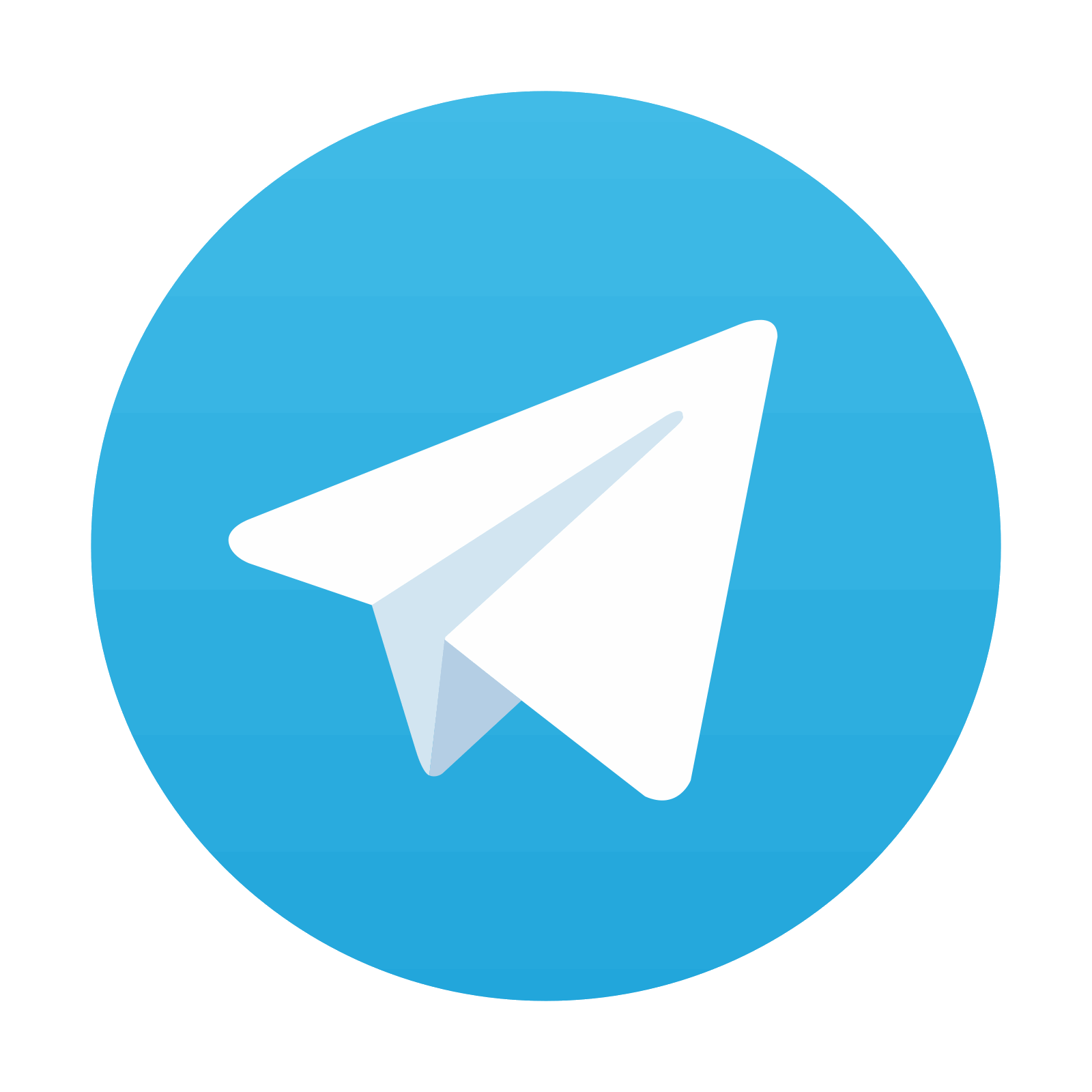
Stay updated, free articles. Join our Telegram channel
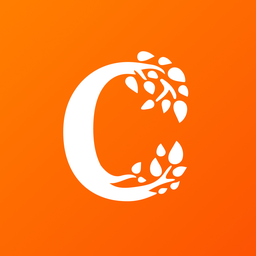
Full access? Get Clinical Tree
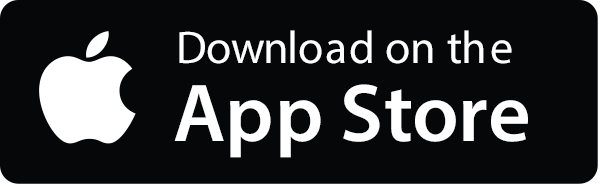
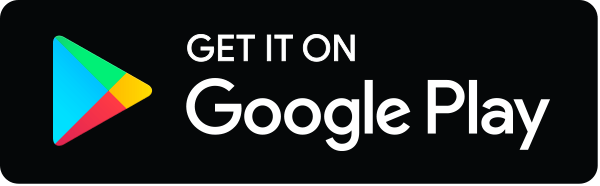