Energy and Protein Nutrition Management of Transition Dairy Cows
Ian J. Lean, BVSc, DVSc, PhD, MANZCVSa∗, Robert Van Saun, DVM, MS, PhDb and Peter J. DeGaris, BVSc (Hons), PhDc, aSBScibus, PO Box 660, Camden, New South Wales 2570, Australia; bDepartment of Veterinary & Biomedical Sciences, Pennsylvania State University, University Park, PA 16802, USA; cTarwin Veterinary Group, 32 Anderson St, Leongatha, Victoria 3953, Australia. E-mail address: ianl@sbscibus.com.au ∗Corresponding author.
Keywords
Transition
Protein
Skeleton
Antioxidant
Vitamins
Introduction
The periparturient or transition period in the 4 weeks before and 4 weeks after calving is characterized by greatly increased risk of disease.1–3 The last 3 to 4 weeks of gestation are characterized by a period of rapid fetal growth, colostrogenesis and mammary development, and metabolic adjustments favoring mobilization of fat and other nutrients; all in combination with declining dry matter intake (DMI). The first 3 to 4 weeks after parturition features slowly increasing DMI in conjunction with rapidly increasing nutrient losses in support of milk production. During this time rapid mammary tissue growth continues as well as hypertrophy of key metabolic and digestive organs. Metabolically, the cow is in a state of nutrient reserve mobilization, primarily adipose and labile protein but also bone. A critical adaptation is to stabilize homeostatic control mechanisms of key nutrients including glucose and calcium in support of lactation. These adaptations to the demands of the fetus and lactation are a process termed homeorhesis.4 Homeorhetic processes are the long-term adaptations to a change in state such as from being nonlactating to lactating or nonruminant to ruminant and involve an orchestrated series of changes in metabolism that allow an animal to adapt to the challenges of the altered state.
Diseases that result from disordered homeorhetic change reflect disorders in homeostasis, in other words, these are failures to adapt that result in shortages of nutrients that are vital for existence. These conditions are often interrelated2,5,6 and include
• Hypocalcemia and downer cows
• Ketosis, fatty liver, and pregnancy toxemia
• Retained fetal membranes/metritis
Grummer7 stated that “If transition feeding is important, then perturbations in nutrition during this period should affect lactation, health and reproductive performance.” There is now a substantial body of evidence clearly confirming that the transition period represents a brief but critically important period of time in a cow’s life when careful manipulation of diet can have a substantial impact on subsequent health and productivity. The aims of transition can be summarized in the establishment of 4 freedoms from the disorders outlined in Table 1.8
Table 1
Condition | Detail |
Macromineral deficiency | Mainly refers to calcium, magnesium, and phosphorus. Milk fever and grass tetany (hypomagnesemia) can result from a conditioned deficiency whereby excess potassium reduces the capacity of the cow to maintain stable blood concentrations of calcium and magnesium |
Lipid mobilization disorders | Includes ketosis, fatty liver, and pregnancy toxemia; diseases that are largely influenced by a failure to provide sufficient or effective energy sources around calving |
Immune suppression | Often associated with lack of energy or protein intake; micronutrients are often involved including copper, selenium, zinc, and vitamins A, E, and D |
Ruminal disruption | Cows are vulnerable to changes in diet resulting from lower feed intake, poor quality silages, and rapid introduction of concentrates after calving |
By achieving these goals, minimizing the loss of body protein before calving, and enhancing mammary development in the periparturient period, milk production will increase in the subsequent lactation. Although transition cow nutrition research results are not consistent across studies, milk production responses relative to controls are in the order of 500 to 1000 kg of milk per lactation with improved transition cow nutrition and management. Targets for the various disease conditions encountered during transition are outlined in Table 2 and a framework for understanding the effects of transition and longer-term nutrition on reproduction is provided in Fig. 1.
Table 2
Disease | Target (%) | Alarm Level (%) |
Milk fever | 1 (2 in old cows >8 y) | >3 |
Clinical ketosis | <1 | >2 |
Subclinical ketosis >1 mmol/L (enzymatic assay week 1 after calving) | <10 | >10 |
Retained placenta >6 h | <5 | >7 |
Lameness >2 Specher et al locomotion score (1–5) | <4 when score >2 | >4 when score >2 |
Clinical mastitis | <5 cases/100 cows in first 30 d | >5 |
Hypomagnesemia | 0 | Any |
Calvings requiring assistance | <2 | >3 |
Displaced abomasums (%) | <1 | >2 |
Clinical acidosis (%) | <1 | >1 |
What is the nature of metabolic change around calving?
Changes in hormone metabolism before calving have been well described.4,9 Bauman and Currie4 noted the following adaptive changes to lactation: increased lipolysis, decreased lipogenesis, increased gluconeogenesis, increased glycogenolysis, increased use of lipids and decreased glucose use as an energy source, increased mobilization of protein reserves, increased absorption of minerals and mobilization of mineral reserves, increased food consumption, and increased absorptive capacity for nutrients. Examining the homeorhetic and homeostatic responses to lactation assists understanding of the factors influencing the risk of disease. These responses can be exaggerated or perturbed by release of inflammatory mediators from lipid mobilization, environmental stressors, or subclinical disease conditions increasing postparturient disease risks.10–14
Insulin and glucagon play a central role in the homeostatic control of glucose. There is evidence, however, of insensitivity of cows to insulin in early lactation. The transitory hyperglycemia that occurs at calving does not seem to stimulate insulin. The insulin responses in hypoglycemic and ketonemic cows were less for glucose infusions and feeding than in normal cows, suggesting marked insulin resistance. Metz and van den Bergh15 found that the response of adipose tissue to insulin in the periparturient cow was altered, because insulin addition did not reduce rates of lipolysis in vitro. Lipogenic activities of adipocytes are reduced by about one-third after calving. Glucagon plays a gluconeogenic role in the bovine, but may not stimulate lipolysis to the same extent as in nonruminant species. Thyroid hormone has a lactogenic function either when supplied orally or when injected and has been used in experimental protocols to induce ketosis.16 However, thyroxine levels either decrease or are unchanged after calving.
Fetal Demand for Nutrients
Bell9 reviewed studies examining the nutrient demands of the fetus in late gestation. The fetal requirements for energy, although modest, are demanding in that the requirement for glucose is 4 times greater than that for acetate.
This demand highlights problems with the low energy density/low energy intake of dry cow diets recommended in the past. The fetus may even have an a priori demand for glucose as plasma glucose concentrations decreased in cows treated with sodium monensin before calving, despite the glucogenic action of monensin.17
The fetus also has significant requirements for amino acids, which are used for tissue deposition and oxidation. The fetal requirement for amino acids seems to be 3 times greater than the net requirement for fetal growth9 because of significant oxidation of amino acids in the fetus.
Challenges to successful adaptation
Reduced Dry Matter Intake
Periparturient disease conditions are associated with decreased DMI, and feed intake is a critical determinant of health and productivity in the dry period. Feed intake and nutrient density of the diet determine the availability of nutrients to the cow and rapidly developing fetus. Grant and Albright18 reviewed the feeding behavior and management factors during the transition period for dairy cattle and found that feed intake decreased by about 30% during the week before calving.
Factors that influence feed intake include social dominance, digestibility of the diet, access to feed, and palatability of the feed.18 Cows and sheep with higher body condition scores have lower DMI after parturition,19,20 and lower DMI has been noted immediately after calving and before calving21 for cows with clinical ketosis.
Stephenson and colleagues17 found that plasma 3-hydroxybutyrate (BHB) concentrations increased from 35 days before calving, in association with increased plasma free fatty acid concentrations (NEFA). Similar increases have been observed in other studies. It is unclear whether BHB and nonesterified fatty acid (NEFA) levels increase as a result of lower DMI, changes in the nutrient density of the diet, or increased fetal demand.
Providing access to feed for more than 8 hours per day and maintaining adequate availability and nutrient density of feed, controlling dominance behavior by grouping and providing adequate feed access and controlling body condition to an ideal of approximately 3.5 on the 5 point scale22 reduce the risk of inadequate nutrient intake. In particular, the use of more digestible forages with lower slowly digestible fiber content allows greater DMI. Considerations around grouping of cattle need to be tempered with an understanding that movement of cattle between groups results in activity to establish new social hierarchies.23–25
The effect of greater DMI was demonstrated by force feeding periparturient cows through a ruminal fistula.26 Cows that received more feed had less hepatic lipid accumulation and higher milk production after calving. The higher milk production resulted from greater postpartum feed intake and a highly significant positive correlation between precalving and postcalving feed intake was identified.26 DMI before calving seems to be an important determinant of production after calving.
Impact of Lipid Mobilization on Liver Function
Reynolds and colleagues27 measured glucose flux across portal-drained viscera over the transition period, finding minimal net change but a 267% increase in total splanchnic tissue output of glucose. This dramatic increase was almost exclusively a result of increased hepatic gluconeogenesis. Increased hepatic protein synthesis9 and greater oxygen consumption27 during transition are consistent with increased hepatic gluconeogenesis. The liver seems to be able to better synthesize glucose from propionate at 21 days postpartum compared with 1 day postpartum or 21 days prepartum.28 The volatile fatty acid (VFA), propionate, is the primary source (50%–60%), of glucose but other substrates must be used to maintain glucose production, including lactate (15%–20%) from placental metabolism and skeletal muscle glycolysis, amino acids (20%–30%) from skeletal muscle catabolism and dietary absorption, and glycerol (2%–4%) released from adipose tissue lipolysis.27,29
Overton30 examined the effects of lipid mobilization on liver function. Increased tissue mobilization increases the flux of NEFAs to the liver for oxidation and increases the need to export some of these back to peripheral tissues as ketones. The liver may not be able to reexport sufficient of these and accumulates fat in hepatocytes. The implications of this accumulation are that the rates of both gluconeogenesis and ureagenesis may be impaired.31 Strang and colleagues31 found that hepatic ureagenesis was reduced 40% through exposure of liver cells to NEFAs, which resulted in increased triglyceride accumulation similar to that of cows after calving. Triglyceride accumulation in the liver around the time of calving has been recognized for many years.7,32
Excessive lipolysis results in a greatly increased blood NEFA concentration and greater hepatic triglyceride accumulation and is associated with a higher risk of 1 or more periparturient diseases.7,33–36 Any factor resulting in greater energy demand (eg, twin pregnancy, cold stress), lower energy intake (eg, poor feed quality, reduced feed availability, heat stress) or both decreases energy balance and ultimately blood NEFA concentration.7
Energy and protein metabolism: opportunities and limitations to improving performance
The demands for amino acids and glucose by the fetoplacental unit, and amino acids, glucose, and fatty acids by the mammary gland, particularly during stage 2 lactogenesis,9,37 combine with a lowered potential DMI immediately before calving38 to place the cow at great risk of mobilizing significant amounts of body fat and protein.
Energy Reserves: Body Condition
The body condition score (BCS) is a major determinant of the calving to first estrus interval; cows in higher body condition display estrus earlier.19 Improved prepartum nutrition to increase BCS to moderate or better at calving was generally associated with increased milk production in dairy cows.39–41 Excessive BCS (>3.75, scale of 1 to 5) in late pregnancy induces lower DMI during late pregnancy and early lactation resulting in greater negative energy balance in early lactation, as shown by excessive reduction in BCS (>1).42 Westwood and colleagues43 identified several factors that significantly influenced the display of estrus at first and second ovulation. Higher body weight of cattle before calving and postcalving appetite were significant factors that increased estrus display. Measures of metabolites in blood that reflected a better energy balance, including cholesterol concentrations and the ratio of glucose to 3-hydroxybutyrate, were also associated with greater display of estrus at ovulation.
Energy Intake: Carbohydrates
The estimated energy balance after calving improves with increased energy density of the prepartum ration.44–46 These improvements have been associated with trends toward increased milk production, lowered milk fat percentage, and significant increases in protein percentage and yield.45 The effect of increased energy density of the prepartum diet, in particular increased fermentable carbohydrate concentration, may in part be mediated through increased development of rumen papillae in response to increased VFA production.47 The increased absorptive capacity of the rumen may have reduced the risk of VFA accumulation and depression of rumen pH and the subsequent risk of acidosis in response to the feeding of high concentrate diets postpartum. Because adaptation and development of rumen papillae takes between 3 and 6 weeks,48,49 the benefit of increasing exposure to a prepartum diet high in fermentable carbohydrate is likely to be curvilinear. There are also likely to be benefits associated with the prepartum adaptation of the rumen microflora to postpartum diets high in concentrates.2 However, there is a challenge in controlling the risks of metabolic disease, particularly lipid mobilization disorders should cows become overconditioned. Overconditioning, however, is more a function of lactation diets than those in the dry period.
Acidosis
Part of the challenge is to provide diets appropriate to preparing the rumen for feeds of greater energy density, but controlling weight, particularly lipid, gain. Adaptation to feeds of higher energy density is important; in almost all production systems, there is a marked increase in exposure to starches and sugars at the time of calving. These increase the risk of acidosis, a relatively poorly understood condition. There are significant recent reviews and articles that advance our understanding of acidosis and the definition of acidosis. Although measures of rumen pH are often used for diagnosis, inconsistencies in cut-off thresholds that define acidosis severity have created confusion in the definition of acidosis.50–52 Studies and definitions of acidosis based on area under the curve estimates of pH are likely sound; these have largely been conducted on limited numbers of fistulated cattle, therefore production outcomes such as milk production, weight gain for beef cattle, or lameness have not been associated with the cut-off points. There is a lack of studies that relate ruminal conditions to outcomes of acidosis or risk factors for acidosis, apart from diets deliberately designed to challenge rumen function; however, a few studies have provided a more detailed examination of acidosis based on large numbers of cattle. Bramley and colleagues,53 Morgante and colleagues,54 and O’Grady and colleagues55 sampled 800, 120, and 144 head of dairy cattle, respectively, and investigated associations between diets and outcomes. All 3 studies provided similar findings of associations between low ruminal pH and a ruminal environment in which the levels of total VFA were increased, but propionate and valerate were particularly increased. Bramley and colleagues53 found that approximately 10% of cows were in the group characterized by high ruminal concentrations of propionate, acetate, butyrate, valerate, and lactic acid, and low ammonia and pH, compared with other groups of cows. The least predictive variables for this group were pH and lactic acid concentrations and the most predictive were propionate and valerate concentrations. These cows had lower milk fat percentages and herds with a high prevalence of acidotic cows had a higher prevalence of lameness and diets lower in neutral detergent fiber and higher in nonfiber carbohydrates, suggesting that the categorization of these cows was sound.
It is unsurprising that pH was a poor predictive variable for acidosis in the field; the rumen is not homogeneous. Samples obtained by stomach tube, probably from the dorsal sac of the rumen, differ from those obtained using rumenocentesis by approximately 0.5 pH and are poorly related with r2 of 0.2.53 Golder and colleagues56 examined the cut-off points for optimal sensitivity and specificity of use of rumen pH obtained using stomach tube or rumenocentesis in predicting acidosis. The optimal cut-off points for prediction of acidosis were less than 6.6 and less than 6.0 for stomach tube and rumenocentesis pH, respectively; however, neither provided a satisfactory test for acidosis, which was defined using the method of Bramley and colleagues.53 The stomach tube pH had a sensitivity of 0.80 and a specificity of 0.76 and rumenocentesis pH had a sensitivity of 0.74 and a specificity of 0.79. It is likely, however, that testing groups of cows for pH less than 5.5, as advocated by Garrett and colleagues,57 provides a satisfactory indicator of acidosis at the herd level.
There are marked substrate differences in the risk of lactic acid generation and VFA production.51,56 Simply, VFA and lactic acid formation is much more influenced by rapidly fermented substrates such as sugars rather than starches. Therefore, inclusion rates for starch and sugars should be considered separately; however, the recommendations in Table 3 are preliminary.
Table 3
Targets for diets: far off, transition, and fresh cows (first 40 days)
aEnergy intake and content that is desirable varies with body condition.
Protein Reserves: Body Condition
The importance of mobilized tissue protein as a source of amino acids for mammary metabolism and gluconeogenesis may be relatively small over the period from calving to peak lactation,58 but is important in the first 1 to 2 weeks of lactation.9 A reduction in skeletal muscle fiber diameter of 25% was observed immediately after calving59 and a decline in the ratio of muscle protein to DNA was found in ewes during early lactation.60 These findings support the concept that skeletal muscle is an important source of endogenous amino acids in early lactation. This hypothesis that improved protein and energy balance improves subsequent production is supported to some extent by the trend toward proportionally higher milk and protein yields in response to increasing days of exposure to a BioChlor-based (Church and Dwight, NJ) prepartum transition diet in younger cows, which are likely to have a greater energy and protein requirement to support growth (DeGaris, personal communication, 2010).
Estimates of body protein reserves mobilized at calving are 25% to 27% of total body protein in a dairy cow, approximately 10 to 17 kg total.61–63 Belyea and colleagues61 noted that there was a significant variation in the abilities of cows to mobilize protein. Bell9 estimated that a metabolizable protein (MP) deficit over 23 days after calving would be nearly 7 kg without accounting for gluconeogenic costs, and 12.5 kg with gluconeogenic costs included. These values seem consistent with previous studies. If 10 kg of protein was lost from muscle, this would equate to a loss of around 60 kg of muscle mass.
The proteins and ultimately amino acids mobilized are used for mammary milk protein synthesis and for gluconeogenesis in the liver to support lactation. It seems that the rates of mobilization of fat and protein are similar,64,65 but there has been little recent work on the amounts of labile fat and protein in body tissue despite this being an important area of physiology. Given the amounts of body weight lost after calving, especially in cows of high body condition, we can be confident that the lipid reserves of these cows exceed the protein reserves. Vandehaar and St Pierre66 highlighted the partitioning of energy to body weight observed by Oba and Allen67 when lower neutral detergent fiber (NDF) diets were fed. It can be hypothesized that cattle exposed to such diets and achieving higher BCS will have greater start-up milk, but the internal flux of nutrients provided from tissue mobilization has a higher ratio, and almost certainly greater amounts of lipid compared with protein.
Protein Intake Before Calving
Studies investigating optimal concentrations of dietary protein in prepartum diets have focused on crude protein (CP) content,68–71 rumen degradable or rumen undegradable fractions in the diet,69–72 but have not considered in depth the potential for ruminal microbial protein synthesis or MP balance. It has been suggested that by increasing prepartum protein body tissue reserves, the transition cow can better use these reserves after calving to support lactation and minimize metabolic disorders,7,73 an effect possibly mediated through increased labile protein reserves. To examine the data in a systematic way, the authors conducted a meta-analysis of studies that have altered the protein content of feeds before calving. Briefly, a literature search of databases was conducted and the diets from 11 studies containing 26 comparisons were extracted. The following information was extracted: authors, year of publication, journal, study design, control and test protein levels, days before calving that the trial started and days in milk at trial end, rumen undegradable percentages, breed, number of milkings per day, feeding type, housing, parity, number of cows in control and treatment groups, DMI, milk yield (kg/cow/d), milk fat yield (kg/cow/d), milk fat percentage, milk protein yield (kg/cow/d), milk protein percentage, fat corrected milk and level corrected for energy corrected milk, average body weight, and measures of variance (standard error).
Fig. 2 displays a Forest plot of milk production results. In this plot, studies are identified and the size of the box is proportional to the weighting of the study within the meta-analysis. The extremities of the horizontal lines represent the 95% confidence intervals of the results for each comparison and diamonds represent the overall estimates using a fixed effect model using the inverse variance method (I-V Overall), and a random effects model using the inverse variance method of DerSimonan and Laird (D + L Overall). The results were homogeneous but were not significant, indicating that milk production was not increased with increased CP in the precalving diet. Further work is needed to evaluate these responses in terms of MP. A preliminary meta-analysis8 found no relationship between MP yield and subsequent milk production, however the current database is larger and this matter will be revisited. A key consideration is that simply increasing CP may not increase MP and increasing the fermentability of the diet with starch may yield more MP than perhaps that achieved by increasing CP or even undegradable protein.
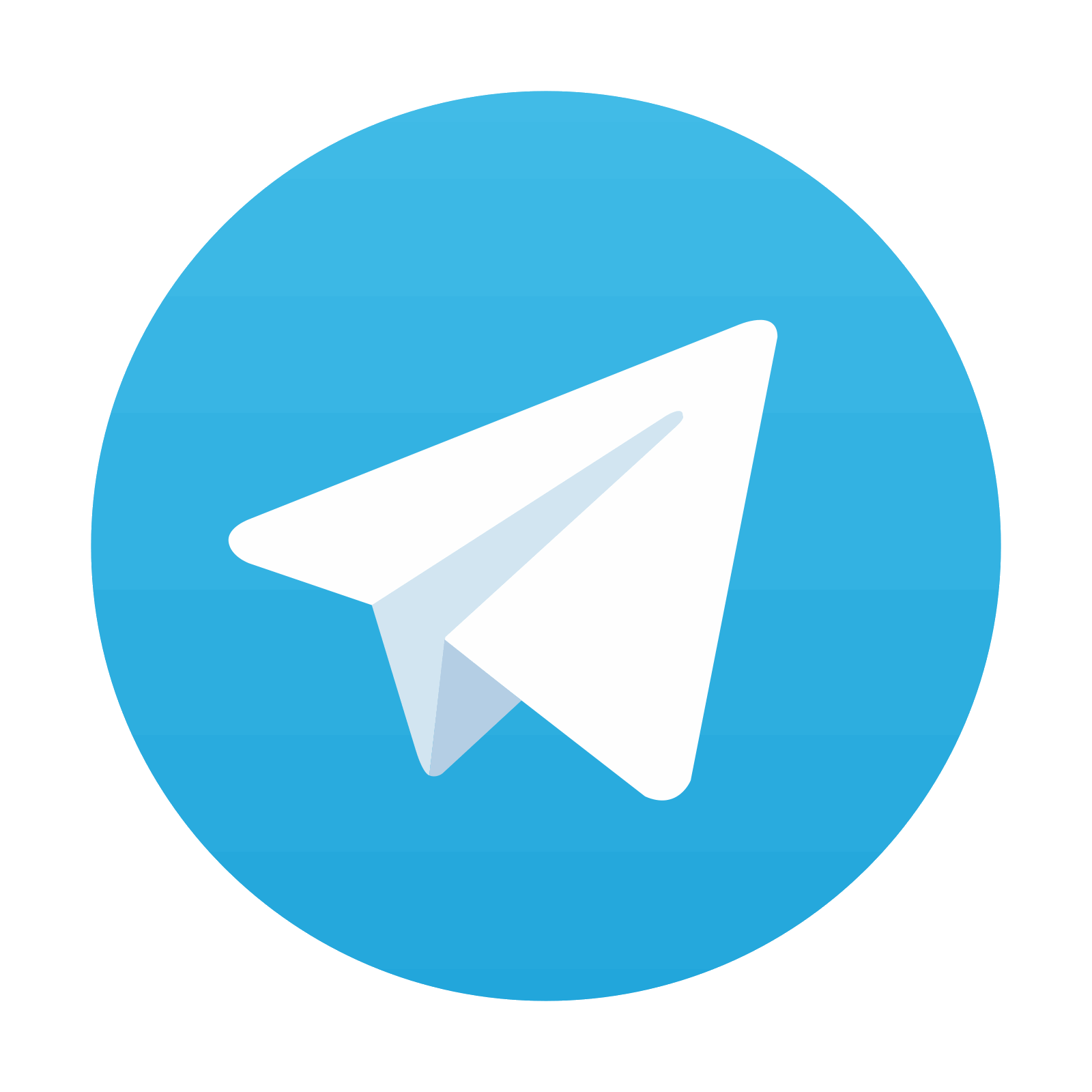
Stay updated, free articles. Join our Telegram channel
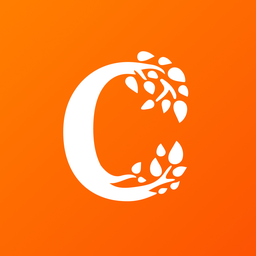
Full access? Get Clinical Tree
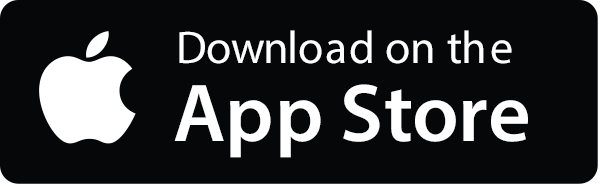
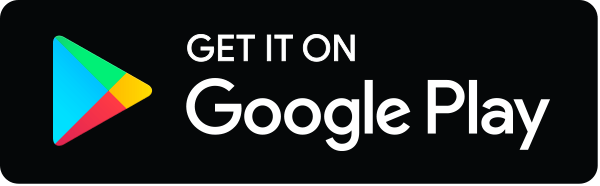