Fig. 1.
Cortical and subcortical areas of the saccadic eye movement system of the monkey. Top: medial view; bottom: lateral view. ACC anterior cingulate cortex, DLPFC dorsolateral prefrontal cortex, FEF frontal eye fields, LIP lateral intraparietal area, SC superior colliculus, SEF supplementary eye fields.
Much of our knowledge regarding the detailed neural mechanisms underlying the control of saccadic eye movements has been gained via recordings of the discharge characteristics of single neurons in rhesus monkeys trained to perform specific oculomotor tasks. Methods for extracellular recordings of single units in conscious animals were first developed by Hubel (9) and Jasper (10), and extended to include the trained, behaving, rhesus monkey by Edward Evarts (11), in his pioneering studies of the neural basis of movement control in the limb motor system. Single neuron recordings in the behaving primate are a powerful, and arguably the most direct approach to understanding the neural basis of behavior, since this method allows the observation of direct correlations between neuronal discharges and the specific components of behavior isolated by the experimental task being performed by the animal.
The utility of this approach in oculomotor control in general, and the cortical control of saccades in particular, is well illustrated by investigations of the role of the FEF in oculomotor control. Early studies of electrical stimulation of the cortex by Ferrier (12) and others (13–16), revealed that stimulation of the frontal lobes of monkeys resulted in contralateral deviations of the eyes. Similar findings were subsequently obtained in humans (17, 18) and together, these results were generally accepted as evidence for a frontal eye field specialized for the initiation of eye movements. This “classical” view was subsequently challenged on the basis of single neuron recordings in the FEF of conscious monkeys, which demonstrated that neurons in this region increased their rate of discharge after rather than prior to the initiation of voluntary but untrained eye movements (19, 20). This discrepancy in findings was resolved by a series of investigations revealing a role of the FEF in control of goal-directed or purposive eye movements by combining recordings of single FEF neurons with strictly controlled oculomotor tasks. These studies demonstrated visual responses (21, 22) (and saccade-related discharges occurring prior to saccade initiation (23)) in FEF neurons. A direct role of FEF neurons in saccade initiation was subsequently demonstrated in single unit studies of monkeys performing the saccade countermanding task (24, 25), and it has been shown that this function is mediated by the connection between the FEF and SC (26).
As the saga of the validation of the classical view of the FEF in saccade generation shows, dissecting the neural circuitry involved in the cortical control of saccadic eye movements requires the combination of strictly controlled behavioral tasks and physiological methods for recording the activity of single neurons. Further, from the anatomy of the saccade network, it is clear that saccade control depends not only upon local processes within the microcircuitry of a cortical area, but also the neural signals sent between nodes of the saccade circuit. This includes connections between not only cortical areas, but also those between cortical areas and subcortical structures with a direct role in saccade initiation, such as the SC. Indeed, as Evarts and colleagues (27) have stated:
It should be appreciated that, insofar as the rest of the brain is concerned, the function of the cortex is mediated by its projection neurons. Only by influencing its subcortical targets…can the cortex affect behavior, either directly or indirectly via other cortical areas (p. 64).
A potentially fruitful approach to understanding the cortical control of saccadic eye movements is to employ a neurophysiological technique that permits investigation of the circuit properties of the saccade system. The technique of antidromic activation enables the identification of the cortical or subcortical projection targets of single neurons in the awake animal, and, when combined with behavioral tasks, a functional characterization of their response properties.
When a neuron’s axon is electrically stimulated, an action potential is evoked which travels backward along the axon and invades the soma. This stimulation-evoked action potential can be observed in extracellular recordings (see Fig. 2). By recording the activity of single neurons in a given brain area, and applying electrical microstimulation to that area’s projection target, neurons sending a direct axonal projection to the target area can be identified. Because electrical stimulation can excite neurons either antidromically or transynaptically, and because no single criterion is diagnostic of antidromic activation, several criteria must be met by evoked action potentials to distinguish direct, antidromic responses from those evoked indirectly via intervening synapses (28–30). First, the latency between onset of stimulation and onset of the evoked action potential should be nearly constant at threshold stimulus intensity. Latencies of action potentials evoked transynaptically typically vary considerably due to variability in the duration of the synaptic processes intervening between stimulation and response, while those of antidromic action potentials are affected only by changes in the excitability of the membrane potential of the stimulated neuron (29, 31). Second, the threshold stimulus intensity required for evoking an action potential should remain stable, and increases in the intensity of stimulation should result in minimal changes in response latency. Synaptic activation typically results in variations in threshold intensity and large reductions in response latency as stimulus intensity is increased (28, 29). The third and most convincing criterion for antidromic activation is the collision test, which determines the presence of collision between spontaneously occurring and evoked action potentials (32–35). When a spontaneous action potential traveling along an axon in the forward, orthodromic direction encounters an antidromically evoked action potential traveling along the same axon in the opposite direction they will collide and both will be abolished (36). The collision test exploits this to establish antidromic activation. To apply the test, the occurrence of a spontaneous action potential in a neuron under study is used to trigger application of electrical stimulation of the target region of interest. If the spontaneous, orthodromic action potential, and the stimulation-evoked antidromic action potential are traveling along the same axon they will collide and no evoked action potential will be observed provided that both are traveling along the same axon at the same time. The time interval within which a collision is expected is referred to as the collision interval, I. With respect to the interval between spontaneous spike occurrence and delivery of stimulation, this interval may be defined as:
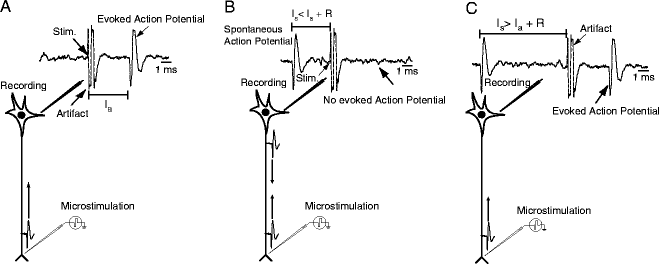
where L is the antidromic latency – the duration between stimulation onset and onset of the evoked antidromic action potential, and R is the refractory period of the axon. If the neuron under study is activated transynaptically, the orthodromic and antidromic action potentials will not collide, and a stimulation-evoked action potential will be observed. Neurons failing to meet any of the criteria noted above are disqualified from consideration as direct projection neurons.
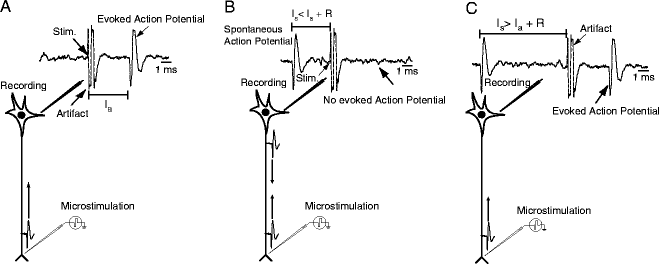
Fig. 2.
Schematic representation of experimental method for antidromic activation. (a) Waveform depicting activity showing artifact caused by microstimulation and stimulation-elicited action potential at the antidromic latency I a. (b) Schematic representation of collision test. Spontaneous action potential triggers microstimulation pulse. If the stimulation latency I s is shorter than I a plus the refractory period R, the two action potentials will collide and no action potential will be observed. (c) Same as (b), but the stimulation latency I s is longer than I a plus the refractory period R. Therefore, microstimulation elicits an antidromic action potential.

The technique of antidromic activation possesses characteristics advantageous to the study of the cortical control of eye movements. By identifying neurons projecting from one region of the oculomotor circuit to another, the technique enables an evaluation of the output signals of a given area. This approach has been employed to investigate signals sent between cortical areas and the superior colliculus; DLPFC to SC (37, 38), LIP to SC (39), FEF to SC (40–43), SC to FEF (44, 45). If the response properties of neurons in the target region are known, antidromic identification allows a direct comparison of output signals from one area to the target structure of interest, and the signals present within the target region. This approach has been applied to establish the progression of visual signals present in area LIP to more motor-related responses in the SC (46). Characterization of neural signals carried by parallel cortico-cortical and cortico-subcortical pathways may also be investigated. For example, Ferraina et al. (47) identified LIP neurons projecting to the FEF or SC and established a visual bias of signals in cortico-cortical but not cortico-tectal pathways. Antidromic activation may also provide a means for investigating the local circuitry of cortical areas by providing supporting evidence for the classification and functional assessment of different neuronal types within a cortical region (48, 49). Because cortico-tectal neurons are located exclusively within layer V (50), identified neurons may also provide a landmark regarding the laminar location of a neuron under study.
Studies aiming to derive a mechanistic understanding of the microcircuitry of the saccade system promise to provide further insight into disruptions in neural processing occurring in a variety of movement disorders, as many can be characterized as the result of disruptions in the function of neural circuits. Here, we aim to provide a basic guide to the study of neural circuits involved in the control of saccadic eye movements in the rhesus monkey model. We begin by briefly discussing the choice of the rhesus macaque as an animal model of the human oculomotor system, some typical behavioral paradigms, then proceed to application of experimental methods including training techniques and antidromic identification.
1.2 The Animal Model
The rhesus macaque has long been the animal model of choice for investigations of the neural basis of oculomotor control. The selection of this model has been based on several criteria. First, the overt oculomotor behavior of humans and rhesus macaques is strikingly similar. Both species produce saccadic and smooth pursuit eye movements with only minor quantitative differences (51). Further, rhesus monkeys can be trained on sophisticated behavioral paradigms, and their performance in tasks such as saccade countermanding (52) and the antisaccade task (53) is similar to that of human subjects. Second, there are substantial structural similarities between the oculomotor systems of humans and macaques. In humans and other primates, the SC contains a map of the contralateral visual field, while in other mammalian species the SC contains at least some representation of both ipsilateral and contralateral visual fields (54). There are also dense projections from frontal cortical areas to multiple layers of the SC in primates (55, 56), which are absent in non-primate species (57). Finally, putative homologies of many electrophysiologically defined areas of the saccade network in the rhesus monkey brain have been established in the human brain using comparative high-resolution fMRI (58–61) and cytoarchitectonic (62, 63) analyses. For example, a homologue of the monkey FEF has been localized to the junction of the superior precentral sulcus with the superior frontal sulcus (64–66). Detailed discussions of the primate model have been provided (67, 68).
1.3 Behavioral Tasks
A range of oculomotor tasks have been designed to investigate the control of saccades (Fig. 3). Such tasks range from simple visually-guided saccade tasks, used to investigate basic processes of saccade control and the responses of single neurons to visual stimuli and saccades, to the antisaccade paradigm, which requires more sophisticated control mechanisms in the form of suppression of a response to a suddenly appearing visual stimulus and a voluntary saccade to the opposite location. Task performance is maintained by providing fluid reinforcement for correct responses. Behavioral indices of performance in these tasks include saccadic reaction time (SRT), proportion of responses correctly directed to the target location, and accuracy of the saccade endpoint with respect to the target location. Accuracy may be expressed as either variability (referred to as endpoint scatter), or systematic shifts with respect to target location. Saccades falling short of the target location are termed hypometric, while those surpassing the target location are referred to as hypermetric.
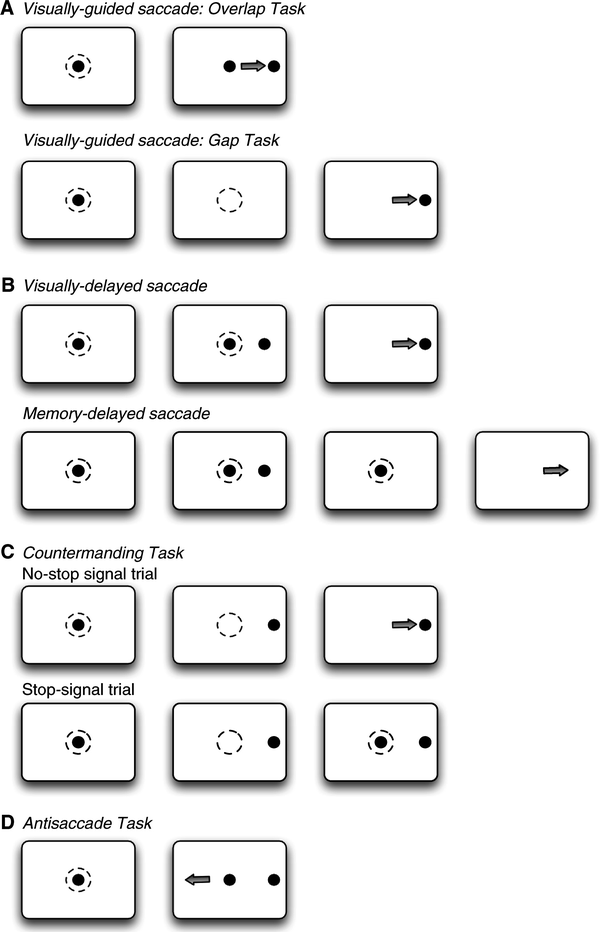
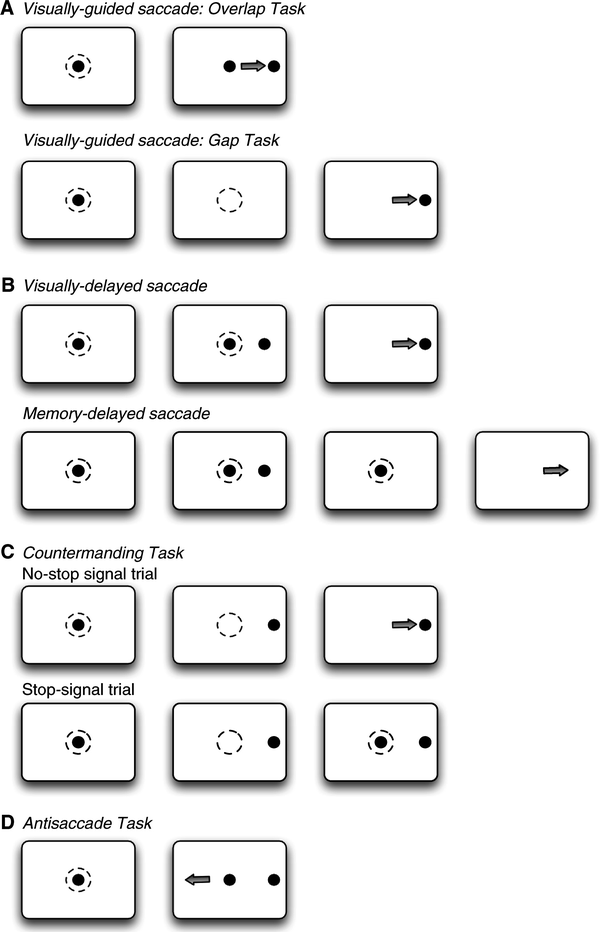
Fig. 3.
Experiment saccade paradigms. The dashed circles indicate where the monkey has to fixate and the arrows indicate the direction of the saccade.
The relationship between performance of these tasks and neural activity is typically investigated by temporally aligning task events, such as visual stimulus onset, or behavioral events, such as saccade onset, with recorded neural activity and carrying out statistical analyses of changes in activity time-locked to those events. Some common paradigms are detailed below.
1.3.1 Visually Guided Saccades
The visually guided saccade task requires the animal to acquire fixation of a central visual stimulus which either (a) disappears a few hundred milliseconds before the appearance of a peripheral visual stimulus (gap task), (b) disappears simultaneously with appearance of the peripheral stimulus (step task), or (c) remains visible while the peripheral stimulus is presented (overlap task) (see Fig. 3a). The duration of presentation of the central fixation stimulus is typically several hundred milliseconds, and is varied in order to avoid anticipatory responses and thus ensure that the saccades are truly visually guided. A well-described observation is the reduction of saccadic reaction times in the gap task compared to the overlap task in healthy human subjects (69) and also in non-human primates (70). In addition to this so-called gap-effect, many subjects also generate saccades with extremely short reaction times in the gap condition. These saccades are termed express saccades and have reaction times between 90 and 120 ms in humans (71, 72), and 70 and 90 ms in monkeys (73, 74). Neural correlates for the gap effect have been found in the FEF (41, 75), DLPFC (76), LIP (77), and the SC (78, 79).
1.3.2 Delayed Saccade Task
This class of tasks inserts a delay between visual stimulus presentation and saccade onset in order to dissociate neural responses related to visual stimulus onset from those associated with saccade initiation (see Fig. 3b). In the visually guided delayed saccade task, animals are required to fixate a centrally presented visual stimulus for a variable period of a few hundred milliseconds, following which a peripheral stimulus is presented. The fixation and peripheral stimuli remain present concurrently, typically for a delay of several hundred milliseconds. Offset of the fixation stimulus serves as a “go” signal instructing the animal to make a saccade to the location of the peripheral stimulus. A variation of this task is the memory-guided delayed saccade task (80), which differs in that the peripheral visual stimulus is presented briefly rather than remaining present for the duration of the delay period. Following the delay, the animal is required to generate a saccade to the remembered location of the visual stimulus. Because it requires a saccade to a remembered location, this version of the task has been used extensively to investigate the neural basis of working memory. In most cortical nodes of the saccade circuit, single neurons exhibit changes in activity time-locked to visual stimulus onset, saccade onset, and sustained delay period activity during this task: LIP (81), FEF (23, 82), and DLPFC (82–84).
1.3.3 Saccade Countermanding Task
This task has been used extensively to investigate the neural basis of saccade initiation in a range of cortical areas including FEF (24, 25), SEF (85), ACC (86), and the SC (87). The saccade countermanding task probes the ability to control initiation of voluntary saccades by infrequently presenting a stop-signal in the context of a standard visually-guided saccade task, and requiring animals to withhold a saccade in response to the stop-signal (see Fig. 3c).
Two types of trials are typically presented. On no-stop signal trials, animals are required to acquire and maintain fixation on a central fixation stimulus for a period of several hundred milliseconds. A peripheral visual stimulus is presented concurrent with fixation offset and the animal is required to saccade to the stimulus location. On stop-signal trials, reappearance of the fixation stimulus serves as a stop signal. At variable durations following its initial disappearance, the fixation stimulus reappears and the animal is required to withhold a saccade. Behavioral performance on stop trials task can be modeled as a race between go and stop processes that accumulate toward a fixed threshold (88), with the process reaching threshold first being the behavior that is expressed. Using this model, it is possible to compute the time required to cancel a planned movement; the stop signal reaction time (SSRT). Neural activity recorded concomitantly with performance of this task must meet two criteria to be considered sufficient for saccade initiation. First, discharge must differ between trials on which a saccade is produced and those on which a saccade is cancelled. Second, such changes in activity must occur before the SSRT. Recordings of single neurons in behaving monkeys have shown that the only cortical area with signals sufficient to control initiation of saccades is the FEF (24, 25). A similar finding has been obtained in the SC (87).
1.3.4 Antisaccade Task
The antisaccade task (4) probes the ability to suppress an automatic saccade toward a flashed peripheral stimulus in favor of a voluntary saccade to its mirror location and has been used extensively to investigate inhibitory control of saccades and the neural correlates of response preparation or preparatory set (89). In a typical antisaccade experiment, animals are required to fixate on a centrally presented fixation stimulus. Upon presentation of a peripheral visual stimulus, they are required to either generate a saccade toward the peripheral stimulus (prosaccade trial) or generate a saccade of equal amplitude away from the peripheral stimulus in the opposite direction (antisaccade trial) (see Fig. 3d). Pro and antisaccade trials may be performed either in blocks of trials or randomly interleaved, and task instructions are typically provided to monkeys via the color of the central fixation point. Monkeys can be trained to perform the antisaccade task (53, 90), and numerous single neuron studies have investigated the roles of cortical areas and the SC in this task (see (89) review).
2 Methods
2.1 Behavioral Training
Rhesus macaques can be readily trained to perform oculomotor paradigms using the method of successive approximations (91). This method involves successively rewarding oculomotor responses that are progressively more similar to the desired response. Fluids such as small amounts of water or fruit juice are typically used as rewards. Training in oculomotor paradigms is undoubtedly facilitated by the fact that saccades naturally occur with high frequency, the result being that animals are able to quickly learn contingencies between oculomotor responses and reward. This technique was first applied to training of oculomotor responses in monkeys by Fuchs and Robinson (51, 92). Oculomotor training requires monitoring of eye movements and precise timing of reward delivery in relation to task events. This can be accomplished using commercially available technologies for eye tracking and computerized behavioral control systems. Successive steps in the training progression are outlined below.
2.1.1 Fixation Training
Since most saccade tasks require the monkey to actively fixate prior to or during the presentation of a peripheral saccade target, this is a necessary first step in training on all oculomotor paradigms. A further benefit of this training is that monkeys readily learn that moving the eyes to and fixating visual stimuli generally results in reward, which facilitates subsequent saccade training. The simplest method for training animals to fixate is to present a small picture of an interesting object on an otherwise blank display. The natural curiosity of the animals will typically result in their looking at this stimulus, and this behavior can then be reinforced by providing reward when the animal’s eyes are on target. Initially, rewards can be delivered when the eyes are on target for only a short time. Over successive responses, the required fixation duration may be gradually increased. Following this, the size of the stimulus and area within which eye position must be maintained may be reduced. Finally, the picture may be replaced with a small fixation spot or cross.
2.1.2 Saccade Training
Once monkeys have learned to fixate a small visual stimulus for a sufficient period of time, they may progress to saccade training. As with fixation training, this stage is facilitated by the natural curiosity of the animals; they will generally saccade toward a suddenly appearing visual stimulus without any specific training. The simplest training method is thus to simply add a peripheral visual stimulus to the fixation training protocol and modify the reward contingency such that the reward is delivered when the animal actively fixates and subsequently makes a saccade toward the peripheral visual stimulus upon its appearance. Initially, the animal may be rewarded for saccades directed to a large area surrounding the visual stimulus. The spatial requirements of this response can be made successively more precise by gradually shifting reward to only those saccades made within a restricted area. In addition, the number of directions and amplitudes at which the peripheral stimulus is presented may be restricted to simply left and rightward directions in the early stages of training, and subsequently progressed.
2.1.3 Specialized Training
Once the monkey is able to reliably make visually guided saccades, training in more specialized tasks may commence. Here, we provide as an example the general approach we have employed to train monkeys to perform the antisaccade task. In this task, the color of the central fixation stimulus serves as a behavioral instruction to perform either a pro- or antisaccade. A first step in training on this task is thus to establish a contingency between fixation color and the required saccade. This can be accomplished by training the animal to follow a simple matching rule. On some trials, a colored fixation stimulus may be presented, followed by two simultaneously appearing peripheral stimuli, one of which matches the color of the fixation stimulus and another that does not. To train the animal to follow a matching rule, saccades made to the peripheral stimulus matching the color of the fixation stimulus are rewarded, while those to the peripheral stimulus of non-matching color are not. For example, a red fixation stimulus may be presented, followed by red and green stimuli at opposite directions and equal distances from fixation. The animal would then be rewarded for saccades to the location of the red peripheral stimulus. On other trials, a green fixation stimulus would be presented, and saccades to the green peripheral stimulus rewarded. It is generally easiest for monkeys to learn this color-matching rule if trials with a given color are presented in blocks of several trials in a row, followed by several trials with the other fixation color. Following this, fixation colors may be presented in a randomly interleaved fashion.
Once monkeys are able to perform this color matching task, antisaccade training may commence. In this phase of training, the luminance of one of the colored peripheral stimuli is gradually reduced until eventually the animal is generating a saccade to a blank location. For example, over a number of days, the luminance of the green stimulus may be reduced but saccades to its location will continue to be rewarded. Because the animal has previously learned a color-matching rule, it will initially make saccades to the red peripheral stimulus when presented with a red fixation stimulus, and the green peripheral stimulus when presented with a green fixation stimulus. As the luminance of the green peripheral stimulus is reduced and it becomes progressively less visible, the animal will gradually learn to make saccades away from the location of the red peripheral stimulus. Once the green peripheral stimulus is no longer visible and the animal is able to reliably make saccades toward the red peripheral stimulus when presented with a red fixation stimulus, and away from the red peripheral stimulus when presented with a green fixation stimulus, the color of the red peripheral stimulus may be gradually desaturated. An animal whose performance has been shaped in this manner will make saccades toward the peripheral visual stimulus (prosaccades) when presented with a red fixation stimulus, and away from the peripheral stimulus when presented with a green fixation stimulus. As with training of color matching, this training may progress more readily when colored fixation stimuli are initially presented in blocks of trials, and randomly interleaved only when animals have obtained a high level of performance.
2.2 Antidromic Identification
2.2.1 Experimental Setup
Neurophysiological experiments using antidromic identification do not require a great deal of specialized equipment that is not generally available in a standard laboratory set up for single neuron recordings in awake-behaving macaques. Commercially available microstimulators and photoelectric stimulus isolation units typically used for microstimulation of the brain should suffice. An oscilloscope that can be triggered by microstimulation onset, and from which voltage traces can be saved is required. No specialized procedures are required to prepare animals for such experiments. Standard recording microelectrodes and microelectrode drives are sufficient.
Because most cortical areas involved in the control of saccadic eye movements project to the superior colliculus, we detail below the steps involved in antidromic identification in the context of an experiment seeking to identify cortical neurons sending a direct projection to the SC – cortico-tectal projection neurons.
2.2.2 Place-Stimulating Electrodes in the Target Region: SC
Because the technique of antidromic identification depends on activation of axons within the target region (30), stimulating electrodes must be placed with care. In this regard, the known anatomy of projections to the SC can be exploited. The majority of axons entering the SC do so via the rostral pole (93), and the probability of antidromically stimulating a cortico-tectal axon is concomitantly greater when stimulation is applied at this collicular site. A similar logic has been employed with regard to antidromic identification of corticospinal neurons, in which stimulation is typically applied at the medullary pyramids, through which all corticospinal axons pass (35). For this reason, it is useful to map the topography of the SC by determining the response fields of single SC neurons and performing microstimulation. Because the intermediate layers of the SC contain a topographic map of saccade direction and amplitude, with smallest amplitudes represented at the rostral pole (94), this region of the SC can be readily identified by observing the direction and amplitudes of the response fields of SC neurons, and the direction and amplitudes of saccades evoked by microstimulation. As the influence of cortical outputs on saccade generation are typically under study, stimulation electrodes should be located in the intermediate layers of the SC. Practically speaking, electrodes should be located at a depth at which saccades can be evoked at the lowest possible stimulation intensity. Stimulation electrodes may be advanced daily, or fixed in place within the recording chamber using epoxy (see Note 1). The latter method has the advantage that multiple stimulation electrodes may be fixed at multiple locations across the SC intermediate layers, thus maximizing the likelihood of antidromically activating an incoming axon.
< div class='tao-gold-member'>
Only gold members can continue reading. Log In or Register a > to continue
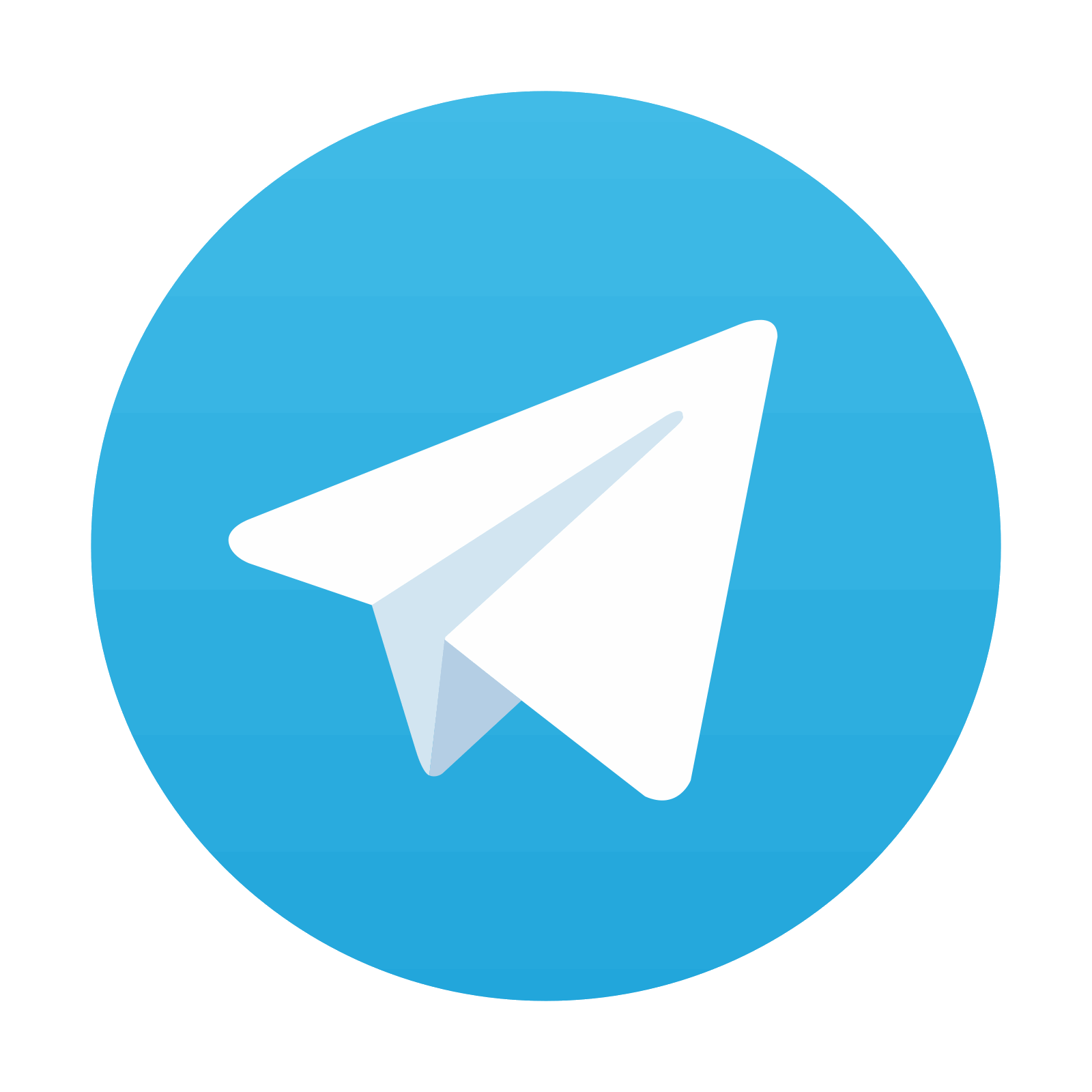
Stay updated, free articles. Join our Telegram channel
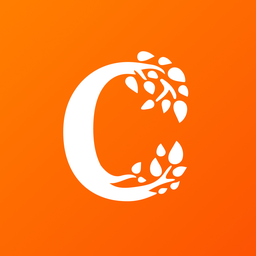
Full access? Get Clinical Tree
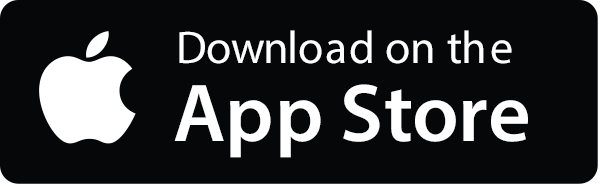
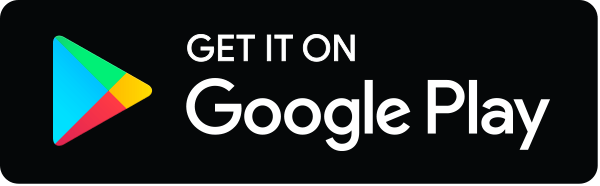