6 Amino acids and protein
Basic properties of proteins and amino acids
Protein and amino acid digestion and absorption
Dietary sources of protein and amino acids
Supplementation of individual amino acids/amino acid derivatives
Protein and amino acid requirements of horses
Methods of assessing dietary protein/amino acid adequacy
Signs of protein/amino acid deficiency and excess
Basic properties of proteins and amino acids
Chemical structure and classification
The general structure common to all amino acids is shown in Fig. 6.1. Each amino acid consists of an α-carbon that is attached to an amino group, a carboxylic acid group and a side chain group, which is unique to each amino acid. There are 21 different amino acids that are a part of mammalian proteins (Fig. 6.2), and these amino acids can be classified based on the chemical properties of the side chain group and on the basis of dietary essentiality.
A common method of classifying amino acids is based on whether or not the animal requires a preformed dietary source of the amino acid or whether the amino acid can be made through the animal’s own metabolic processes in quantities sufficient enough to meet metabolic needs. Unlike bacteria, which have the metabolic pathways to synthesize all of the amino acids de novo, mammals only have the enzymatic ability to synthesize some amino acids. Amino acids that must be provided in the diet, because metabolic pathways for their synthesis do not exist or are insufficient to meet demands, are referred to as indispensable (essential) amino acids, and amino acids that the animal can make through its own metabolic pathways are termed dispensable (non-essential) amino acids. This classification system leaves a gray area for the amino acids where metabolic pathways are present, but under certain circumstances are unable to make sufficient quantities of the amino acids to meet metabolic requirements. These amino acids are termed conditionally indispensable amino acids. In mammals, there are nine amino acids that are classified as strictly indispensable amino acids: histidine, isoleucine, leucine, lysine, methionine, phenylalanine, threonine, tryptophan, and valine. Arginine is often included in this list because in many growing mammals the rate of its synthesis is insufficient to meet the animal’s demands; however, with the exception of obligate carnivores, arginine appears to be made in adequate quantities in mature, healthy mammals, making a dietary source of this amino acid unnecessary (reviewed by Ball et al 2007). Arginine may also become an indispensable amino acid during illness or injury because of the roles that it plays in the immune system function and in the disposal of the excess nitrogenous wastes that are typical of a catabolic state. Other conditionally indispensable amino acids include: tyrosine, which can be synthesized from phenylalanine if sufficient phenylalanine is provided in the diet; cysteine, which can be synthesized from dietary methionine; glycine and proline, which may not be synthesized in sufficient quantities during growth; and glutamine which becomes essential in times of illness or physiological stress. The amino acids widely accepted as being dispensable among mammals are alanine, asparagine, aspartate, glutamate, and serine. Selenocysteine, a relatively “new” amino acid discovery and a critical component in selenoproteins such as glutathione peroxidase, has not been officially classified on the basis of its dietary essentiality. However, because selenocysteine does not readily exist as a free amino acid and its synthesis occurs directly onto the tRNA molecule (Allmang et al 2009), this amino acid appears to be dispensable so long as adequate selenium is available.
Protein synthesis involves the transcription of the cell’s genetic material, DNA, into RNA which is then translated into protein. During translation, the amino acids that are attached to the tRNA interact with the mRNA and adjacent amino acids are covalently joined together by peptide bonds (Fig. 6.3). These covalent peptide bonds are strong and once formed they can only be broken by enzymatic activity or by a combination of low pH and high heat. The amino acid sequence of each protein is unique and determines the three-dimensional structure and the specific function of the protein. For this reason, even a one amino acid substitution in a protein, can have major implications on the structure and subsequently the function of the protein.
Physiological roles of protein and amino acids in the body
There are also several nitrogen-containing compounds that are synthesized from amino acids, but because they are formed through enzymatic reactions rather than through RNA transcription, they cannot be classified as proteins. These nitrogen-containing compounds have a variety of physiological functions. Glutathione (cysteine, glycine and glutamine) is an antioxidant that interacts with free-radicals and protects cells from oxidative damage. Creatine (arginine, glycine, and methionine) is involved in cellular energy metabolism, because when phosphorylated it can be used for the rapid generation of ATP. Carnitine (lysine, methionine) is necessary for the transport of fatty acids across the inner mitochondrial membrane for oxidation and ATP production. Carnosine (histidine and β-alanine) is present in very high concentrations in equine skeletal muscle fast twitch fibers (Sewell et al 1992), where it aids in buffering the lactic acid produced during glycolysis. The synthesis of the purine and pyrimidine bases, the molecular constituents of DNA and RNA, requires the nitrogen and/or carbon atoms from several amino acids: glutamine (purines/pyrimidines), aspartate (purines/pyrimidines), and glycine (purines).
Key Points
• Amino acids can be classified based on their chemical properties and on whether or not a dietary source must be provided
• Important functions of protein in the body include structural, enzymatic and hormonal roles, transport of nutrients across membranes and in the blood, and as a component of the immune system
• In addition to being a component of protein, certain amino acids have additional physiological roles
Protein and amino acid digestion and absorption
General overview of protein digestion
Undigested protein and unabsorbed amino acids pass into the large intestine, where, in other monogastrics, cecal peptidase activity is much lower than in the small intestinal segments (Bai 1993). There has been little research in horses about the microbial digestion of protein; however, microbial protein digestion in the rumen of cattle has been extensively studied and provides insight into the processes that may be occurring in the equine large intestine. In ruminants, proteases associated with the bacterial cell wall begin to degrade proteins into smaller peptide chains, which can then be absorbed by the bacteria, cleaved into individual amino acids and either used for microbial protein synthesis or further degraded to carbon skeletons and ammonia (Wallace 1996). In bacterial cells isolated from the cecal contents of horses, all species of bacteria could use partially digested protein as a nitrogen source, with smaller subsets of cecal bacteria also able to use ammonia and urea as sole nitrogen sources (Maczulak et al 1985). An important distinction must be made between the sites of microbial protein synthesis relative to the sites of protein digestion in ruminants versus monogastrics. In ruminants, the microbial protein is made proximal to the gastric and pancreatic secretions and therefore can be digested by the animal in a similar manner as dietary protein is digested in monogastrics. In horses, however, microbial protein synthesis occurs predominantly distal to the gastric and pancreatic secretions, and this has implications for both the digestion and absorption of these proteins.
Intestinal amino acid transport
In order to be absorbed from the intestinal lumen into the bloodstream, amino acids rely on specific transport proteins, often referred to as transport systems, to be transported across two sets of enterocyte plasma membranes: the apical (or luminal) membrane and the basolateral (serosal) membrane. In mammalian cells, many different amino acid transport systems have been identified and characterized on the basis of the chemical properties of the amino acids they transport, a requirement for metabolic energy, and whether they are sodium-dependent or independent (Broer 2008a). The expression of the various transport system proteins is membrane-specific, with some systems expressed on the apical membrane and others expressed on the basolateral membrane. Although the majority of the transport systems on the apical membrane transport amino acids into the enterocyte, the basolateral membrane has some systems that transport circulating amino acids into the enterocyte and others that transport amino acids out of the enterocyte. Glutamine, for example, is a key energy substrate in the gastrointestinal tract and is extensively taken up from the bloodstream by both jejunal and colonic enterocytes in horses (Duckworth et al 1992). There is also a well-characterized active transport system for di- and tri-peptides, PepT1 (Gilbert et al 2008a). The properties of the common mammalian intestinal amino acid transport system are summarized in Table 6-1 and Fig. 6.4.
Table 6-1 Systems Involved in Amino Acid Transport in the Mammalian Intestine
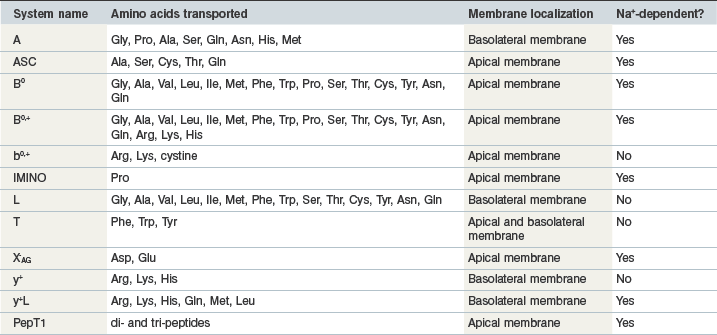
Data from Broer 2008b, Closs et al 2004, Gilbert et al 2008b.
Currently, only a single study exists that has measured the presence and distribution of specific amino acid transport systems in the equine intestine (Woodward et al 2009). This study examined the mRNA abundance of the genes corresponding to the apical membrane system b0,+, the basolateral membrane system y+ and for two genes that encode for the basolateral membrane system L. The b0,+ system mRNA was similarly expressed in the jejunum, ileum, cecum and colon of mature horses, while the gene corresponding to the y+ system was expressed to a greater extent in the small intestinal segments compared to the large intestinal segments (Woodward et al 2009). The mRNA abundance of the medium affinity L transport system was higher in the small intestine than in the large intestine; however, the abundance of mRNA corresponding to the lower affinity L system was higher in the cecum than in the jejunum (Woodward et al 2009). These findings provide a potential mechanism whereby amino acids synthesized by the large intestinal microbes could potentially be absorbed (for further discussion, see below), although additional research is necessary to characterize the distribution of other amino acid transport systems along the equine intestinal mucosa.
The peptide transporter, PepT1, has also been identified throughout the small intestine of several domestic animals including cattle, pigs, sheep and chickens (Chen et al 1999) and in the cecum of chickens (Chen et al 1999; 2002), although at this time, PepT1 distribution has not been characterized in the equine intestinal mucosa. Incubation of the equine jejunal membrane with the dipeptide glycyl-L-glutamine, in vitro, resulted in an increase in current flow through the membrane, suggesting PepT1-like activity in the equine small intestine (Cehak et al 2009). However, in the rabbit, another hind-gut fermenter with a well-developed large intestine, PepT1 mRNA expression was not detectable in the cecum and was much lower in the colon than in the small intestinal segments (Freeman et al 1995), indicating only a limited capacity to absorb peptides in the large intestine.
Controversy exists about the extent to which amino acids can be absorbed from the large intestine of horses and over the last 40 years, a variety of studies have attempted to quantify the possible contribution of microbial amino acids to whole-body amino acid metabolism in horses. By infusing stable and radioactive isotope tracers into the cecum of horses, one study was able to detect the isotope label in the circulating indispensable amino acids (Slade et al 1971), whereas another study could not (Wysocki & Baker 1975). Orally administered isotopes also showed very minimal (<10%) incorporation into circulating indispensable amino acids, and tissue and milk protein (McMeniman et al 1987, Schubert et al 1991), and because the isotope was fed, it is possible that some of these labeled amino acids were made by microbes proximal to the large intestine. The isotope data indicates that only a small portion of circulating and retained amino acids are synthesized by the large intestinal microbes, suggesting impairments in either the digestion or the absorption of microbially synthesized protein. The limitation in the absorption of microbial amino acids is further supported by the observation that the infusion of a large amount of lysine cecally does not result in the increase in portal vein plasma lysine concentrations that occurred when the lysine was administered gastrically (Wysocki & Baker 1975). Furthermore, the serum concentrations of several indispensable amino acids were significantly correlated to the dietary amino acid composition, but not to the amino acid composition of either cecal fluid or cecal microbes, indicating that the large intestine has only a minimal influence on whole-body amino acid status (Reitnour et al 1970).
The in vitro studies provide additional support that there is limited capacity to absorb amino acids across the apical membrane in both the cecal and colonic mucosa. At physiological concentrations, negligible amounts of lysine, histidine and arginine were able to cross the apical membrane in isolated colonic mucosa (Bochroder et al 1994), and although alanine and a leucine analog are able to cross the basolateral membrane of cecal mucosa, there was also no measurable apical membrane transport of these amino acids (Freeman et al 1989, Freeman & Donawick 1991). Ammonia, on the other hand, was readily transported across the mucosal membrane of the proximal colon (Bochroder et al 1994).
To summarize, despite the presence of some apical amino acid transport systems in the equine large intestine (Woodward et al 2009), it appears unlikely that the indispensable amino acids synthesized by microbes in the large intestine are absorbed to a large enough extent to make a substantial contribution to meeting the horse’s amino acid needs.
Digestibility of dietary protein
The term protein digestibility refers to the portion of dietary protein that disappears, and is presumably absorbed, along the length of the gastrointestinal tract. A summary of the different types of digestibility that can be calculated is provided in Table 6-2. Digestibility can be defined as either total tract or prececal, depending on whether the undigested nitrogen is measured in the feces or ileal contents, respectively. Total tract protein digestibility is a measure of how much nitrogen is absorbed throughout the length of the intestine; however, it does not identify the location within the gastrointestinal tract of this digestion or the form (amino acids versus ammonia) that the nitrogen is absorbed in. Based on the discussion in the “Intestinal amino acid transport” section, it appears that the vast majority of amino acids are absorbed prior to the ileum and that most postcecal nitrogen absorption is likely to be largely as ammonia which is only available for protein synthesis if it is first incorporated into dispensable amino acids following absorption. Therefore, prececal protein digestibility (also referred to as ileal protein digestibility) may be a more reliable indicator of the amount of protein nitrogen that is absorbed in a form that is readily available for protein synthesis. Digestibility can also be classified as either true or apparent, depending on whether endogenous losses (microbial protein, enzymes, sloughed cells, etc.) are corrected for in the resulting fecal or cecal output (Table 6-2). Endogenous losses vary based on feed type and are greater with high forage diets than for primarily concentrate diets (Almeida et al 1999b, Farley et al 1995, Gibbs et al 1988, 1996).
Table 6-2 Calculation of Total Tract and Prececal Nitrogen Digestibility
Digestibility terma | Calculation |
---|---|
Apparent total tract nitrogen digestibility | Dietary nitrogen intake – fecal nitrogen output |
Apparent prececal nitrogen digestibility | Dietary nitrogen intake – cecal nitrogen input |
True total tract nitrogen digestibility | Dietary nitrogen intake – (fecal nitrogen output – endogenous nitrogen lossesb) |
True pre-cecal nitrogen digestibility | Dietary nitrogen intake – (cecal nitrogen output – endogenous nitrogen lossesc) |
aProtein is approximately 16% nitrogen, by weight. Therefore, nitrogen digestibility can be converted to crude protein digestibility by dividing by 0.16.
bTotal tract endogenous nitrogen losses have been reported to range from 0.72–9.1 mg nitrogen/kg dry matter consumed (Almeida et al 1999b, Farley et al 1995, Gibbs et al 1988, 1996).
cPrececal endogenous nitrogen losses have been reported to range from 1.8 – 5.8 mg nitrogen/kg dry matter consumed (Almeida et al 1999b, Farley et al 1995, Gibbs et al 1988, 1996).
In mature horses, protein digestibility varies based on the protein source and the forage and concentrate composition of the diet, as summarized in Table 6-3. In the most recent version of the Nutrient Requirements of Horses (NRC 2007), total tract apparent nitrogen digestibility was estimated at 79% and pre-cecal apparent nitrogen digestibility was estimated at 51%. Based on the values summarized in Table 6-3, true and apparent prececal digestibility values are greater for cereal grains and oilseed meals than for forages. Apparent crude protein digestibility in horses increases with crude protein intake because as intake increases, the endogenous losses represent a smaller portion of total intake and make a relatively smaller contribution to total fecal (or prececal) nitrogen losses (Slade et al 1970). For true protein digestibility, there is little effect of protein intake on total tract digestibility, when there is a low to moderate level of protein intake and a constant protein source is used (Farley et al 1995). However, at high levels of protein intake, there may be a decline in both apparent and true pre-cecal digestibility (Farley et al 1995), likely due to limitations in the small intestinal digestive or absorptive capacity. Limited data exists regarding nitrogen digestibility in growing horses; however, in 3–7-month-old foals receiving a 70% concentrate diet, apparent total tract digestibility values of diets containing either milk protein or linseed (flax) meal ranged from ~35–45% (Hintz et al 1971), which is lower than similar high concentrate diets in mature horses (Table 6-3). In growing yearling horses, the apparent total tract digestibilities of 60% concentrate diets were ~80% (Antilley et al 2007), which are comparable to nitrogen digestibility estimates in mature horses (Table 6-3), suggesting that any limitations in nitrogen digestibility at ~6 months of age have been overcome by 15 months of age. In the case of forage-based diets, –
of the total tract true nitrogen digestion and absorption of nitrogen occurs in the large intestine (Table 6-3); whereas for grains more true nitrogen digestion and absorption occurred pre-cecally (> 70%) (Table 6-3).
Table 6-3 Estimates of Apparent and True Amino Acid Digestibilities of Feed Ingredients in Mature Horses
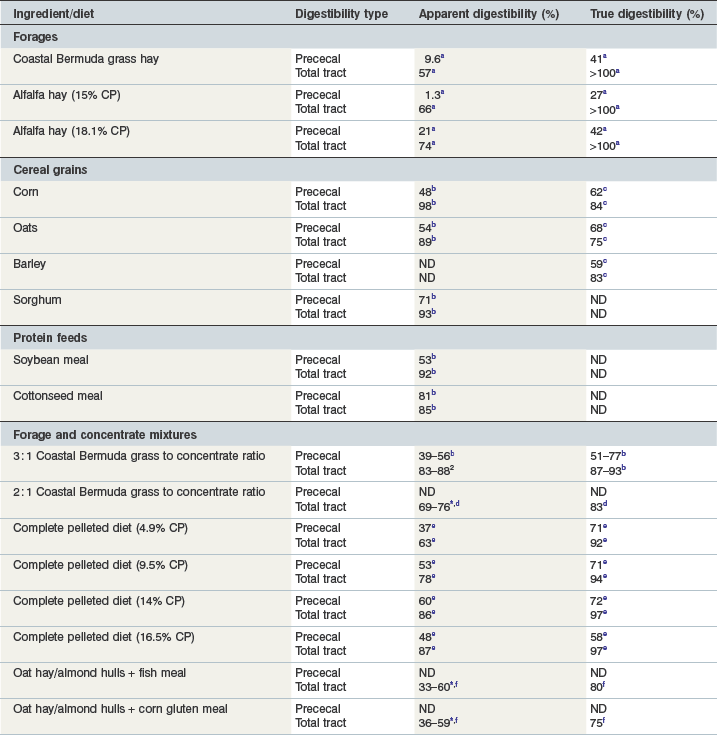
* Apparent digestibility was linearly related to nitrogen intake, with increasing nitrogen intake resulting in increased apparent nitrogen digestibility.
Data from a(Gibbs et al 1988); b(Gibbs et al 1996); c(Rosenfeld & Austbo 2009); d(Freeman et al 1988); e(Farley et al 1995); f(Slade et al 1970).
Although prececal true protein digestibility values should give a reasonable estimate of protein absorbed in the form of amino acids, a better estimate would be to determine the prececal digestibility of each of the individual amino acids. In pigs, the true ileal digestibilities of the amino acids in many feed ingredients have been determined and individual amino acid requirements are provided on a true ileal digestible basis for pigs in a variety of physiological states (NRC 1998). There is very little research in horses that has quantified the prececal digestibilities of the individual amino acids and because of this lack of data it is not possible to estimate prececal digestibilities in the feed ingredients or to estimate the horse’s requirements for prececally digestible amino acids. In growing foals receiving a diet with a 50 : 50 forage-to-concentrate ratio, the apparent digestibility of the amino acids increased with increasing crude protein intake (Almeida et al 1999a). Pre-cecal endogenous nitrogen losses (range: 0.12 to 1.00 mg/g dry matter consumed for threonine and serine respectively) and true pre-cecal digestibilities (range: ~30 to ~100% of intake for alanine and phenylalanine, respectively) were also calculated and are summarized in Table 6-4 (Almeida et al 1999b). These true prececal digestible amino acid values fall within the range of pre-cecal nitrogen digestion that have been reported (Table 6-3) and provide some indication of the extent to which dietary amino acids may be absorbed in the small intestine of horses. It is unknown how the pre-cecal digestibilities of the amino acids vary based on the feed ingredients used or the age/physiological state of the horse and additional research in this area is necessary.
Table 6-4 Estimated Prececal Endogenous Losses and Total Digestibilities of Amino Acids in Growing Foals Receiving a Diet with a 50 : 50 Forage to Concentrate Ratio
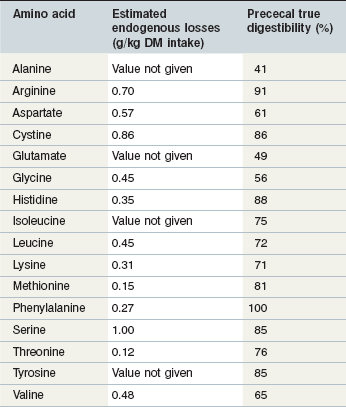
Data from Almeida et al 1999a.
Key Points
• Enzymatic protein digestion begins in the stomach and continues throughout the small intestine, with amino acids as the key end product. Microbial protein metabolism and digestion occurs in the large intestine, with ammonia as the major end product.
• Although luminal amino acid transport systems are present along the entire length of the intestine, it appears as though the majority of amino acid absorption occurs in the small intestine, whereas ammonia is the product absorbed from the large intestine. There is only minimal evidence to support the absorption of amino acids made by microbes in the large intestine.
• The majority of protein digestion from grains occurs prececally, whereas forage protein digestion occurs primarily postcecally.
Dietary sources of protein and amino acids
Milk
During the first few weeks of life, the foal obtains all or most of its dietary protein from milk. The protein concentration in fluid milk declines over the course of lactation. Colostrums may contain 10–19% crude protein on a fluid basis, but the protein concentration in milk is much lower (Martinez et al 1993, Ullrey et al 1966). During early lactation mare’s milk usually contains 2–3% protein (Doreau et al 1992, Gibbs et al 1982, Glade 1991, Ullrey et al 1966). Between 2 and 4 months, the concentration of protein in fluid milk remains relatively constant at approximately 2% (Gibbs et al 1982, Ullrey et al 1966). In late lactation milk protein concentrations may be as low as 1.5 to 1.8% (Davison et al 1991, Mariani et al 2001).
Milk protein is considered a high quality protein source, because of its amino acid composition (Table 6-5) and its high digestibility. A classic study conducted by Hintz and coworkers (1971) demonstrated the importance of protein quality by comparing milk protein to linseed meal as the supplemental protein source in the diets of growing horses. Obviously milk is a source of high quality protein, but after the foal reaches 2–3 months of age most dietary protein will be derived from other sources.
Table 6-5 Amino Acid Composition of Equine Skeletal Muscle and Mare’s Milk
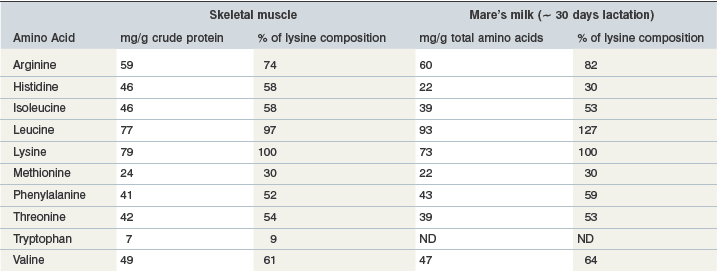
Data from Badiani et al 1997, Davis et al 1994a, b. For both skeletal muscle and mare’s milk, amino acid composition includes both free amino acids and amino acids contained in protein.
Forages
Grasses are usually lower in crude protein than legumes. However, regular application of nitrogen-containing fertilizers to cool-season or warm-season grasses can increase the crude protein content. Well maintained cool-season pastures may contain 14 to 20% CP on a dry matter basis during the growing season (Harris 1997, Hoskin & Gee 2004, Lawrence et al 2006). Common cool season grasses that are used for horse hay or pasture include orchardgrass, Kentucky bluegrass, ryegrass, tall fescue and timothy. Warm-season grasses such as Bermuda grass and bahiagrass are also used for horses particularly in areas where winter temperatures are mild.
Stage of maturity at the time of harvest is one of the most important determinants of forage composition. Crude protein content of forages is highest when the plant is in a vegetative stage of growth and is lowest when the plant is in a late stage of maturity (Table 6-6). Stage of maturity also affects the neutral detergent fiber (NDF) and acid detergent fiber (ADF) in forage. Some of the protein in a forage may be associated with the NDF or ADF fraction, and the proportion of nitrogen found as acid detergent insoluble nitrogen (ADIN) increases with increasing forage maturity (Coblentz et al 1998, Elizalde et al 1999, Gonzalez et al 2001). It has been suggested that subtracting the amount of ADIN in a feed from total nitrogen can be used to estimate available dietary protein in horse feeds (NRC 2007).
Table 6-6 Effect of Stage of Maturity on Crude Protein Content of Forages
%CP | |
---|---|
Alfalfa | |
Pre-bud/bud | 22–26 |
Early bloom | 18–22 |
Midbloom | 14–18 |
Full bloom | 9–13 |
Cool season grasses | |
Vegetative | 16–20 |
Boot | 12–16 |
Head | 8–12 |
Warm season grasses | |
Vegetative | 16–18 |
Boot | 6–8 |
Head | 6–8 |
Adapted from Ball et al 2002.
Factors that influence the total protein concentration of forages will also affect the amino acid concentrations in the forage. In one study, the CP and lysine content of alfalfa hay harvested at three stages of maturity were 22% and 1.2%; 20.9% and 0.95%; and 16.9% CP and 0.64% lysine, respectively, on a dry matter basis (Garcia et al 1995). When the preceding lysine amino acid concentrations are expressed as a percentage of CP, it appears that with increasing stage of plant maturity, lysine decreases as a proportion of total protein, as well as a proportion of the dry matter. However, other researchers have not found a change in amino acid composition expressed as a percentage of CP in forages at different stages of maturity (Vanhatalo et al 2009).
Cereal grains and grain by-products
Cereal grains are incorporated into horse diets for energy, but they do provide protein and amino acids as well. The protein and amino acid composition of commonly used cereal grains are shown in Table 6-7. Within a type of grain, protein and amino concentrations are relatively consistent, and equations have been derived to estimate the amino acid composition of various feeds from their proximate components (NRC 1994). However some caution should be applied to this approach because there may be some differences among cultivars within a species and development of new cultivars has the potential to alter these characteristics. For example, to overcome low levels of lysine and methionine in typical corn varieties, higher lysine and higher methionine varieties have been developed. There are also differences in the protein characteristics of flint, dent, waxy, opaque and floury corn (Larson & Hoffman 2008). A new oat variety developed to have higher fat in the groat and lower lignin in the hull also has altered protein characteristics (Yu et al 2008). As new varieties of traditional cereal grains are used in horse rations, an understanding of their protein characteristics may be important. The crude protein in opaque and floury corn may have less zein than the crude protein in dent corn (Larson & Hoffman 2008). Zein is a type of prolamin protein that encapsulates starch. Higher levels of zein relative to the starch content may reduce the susceptibility of starch to digestion in ruminants (Larson & Hoffman 2008), and could be important in equine nutrition as well.
Both total tract and small intestinal protein digestion are generally greater for grains than for forages and there may be some differences between grain species in regard to the site or extent of protein digestion (Table 6-3). Limited information on small intestinal amino acid digestion by horses is available but reviews of relative digestibilities and bioavailabilities of amino acids in common swine and poultry feeds have been published (Lewis & Bayley 1995, NRC 1994, 1998).
Many byproducts of the grain processing industry are incorporated into horse feeds. Common examples include wheat middlings and wheat bran, but corn gluten feed and rice bran are also considered in this category. In many cases the by-product feed has more crude protein than the parent grain. For example, corn grain has been reported to contain 8.3% CP whereas corn gluten feed contains 21.5% CP (as fed basis; NRC 1998).
As mentioned previously, cereal grains do not contain high quality protein. Consequently, grain by-products often contain moderate or low quality protein, even though they may be relatively high in CP. Therefore the amino acid content of by-product feeds should be considered when they are incorporated into horse feeds, especially if their inclusion reduces the use of ingredients with higher quality protein. Reference values for the amino acid composition of many by-product feeds have been published (NRC 1994, 1998, 2007); however, differences in milling procedures at individual plants may result in variation in protein and amino acid composition of these products. An alternative to using reference values for by-product feeds are prediction equations that have been developed to estimate amino acid composition from crude protein alone or in combination with other proximate components (NRC 1994, 1998).
Seed meals and other protein and amino acid supplements
When oil is extracted from the oil seeds of soybean, sunflower, canola, etc., the remaining seed meal is a high protein by-product that may be used in livestock feeds. Soybean meal is the most commonly used seed meal in horse feeds because it is widely available and it has a desirable amino acid profile. Raw soybeans contain several antinutritional factors that must be inactivated during the manufacturing process. When whole soybeans are incorporated into horse feeds they should be heated or extruded prior to feeding to inactivate the antinutritional factors. Although heat is essential for the production of useful soybean meal, too much heat can damage the protein and reduce amino acid availability. Amino acid availability may also be affected by soybean variety (Baker & Stein 2009). The protein content of commercially available soybean meal is often standardized at either 44% or 48% CP by the addition of soy hulls.
The protein and amino acid composition of many protein supplements have been well characterized (NRC 1994, 1998). The amino acid profile of soybean meal is generally superior to most other seed meals. Cottonseed meal, sunflower seed meal, safflower meal, peanut meal, canola meal and sesame meal are all comparatively low in lysine. Cottonseed meal and safflower meal are also low in sulfur-containing amino acids. When these protein sources are used it may be necessary to balance the amino acid profile using an amino acid supplement. Feed grade sources of several individual amino acids are available. Although amino acids can be synthesized in either the D or the L form, the amino acids that occur in plant and animal tissue are usually found as L-isomers. The ability of animals to use the D form of individual amino acids varies by amino acid and possibly by species or age of animal (Lewis & Baker 1995). The ability of horses to convert amino acids in the D form to the L form has not been investigated, although in other species the D-form of methionine can be converted to the L-form by the enzyme D-amino acid oxidase and is used as efficiently as the L-form in supporting growth (Baker & Boebel 1980, Chung & Baker 1992). However, supplements containing leucine, tryptophan, creatine, histidine, and β-alanine have also been manufactured for horses.
Supplementation of individual amino acids/amino acid derivatives
Branched chain amino acids (BCAA)
Because the BCAA (leucine, isoleucine and valine) are extensively oxidized for energy in the skeletal muscle, the supplementation of these amino acids has been suggested to have beneficial effects on athletic performance by sparing the use of dietary carbohydrates and glycogen (Harris & Harris 2005
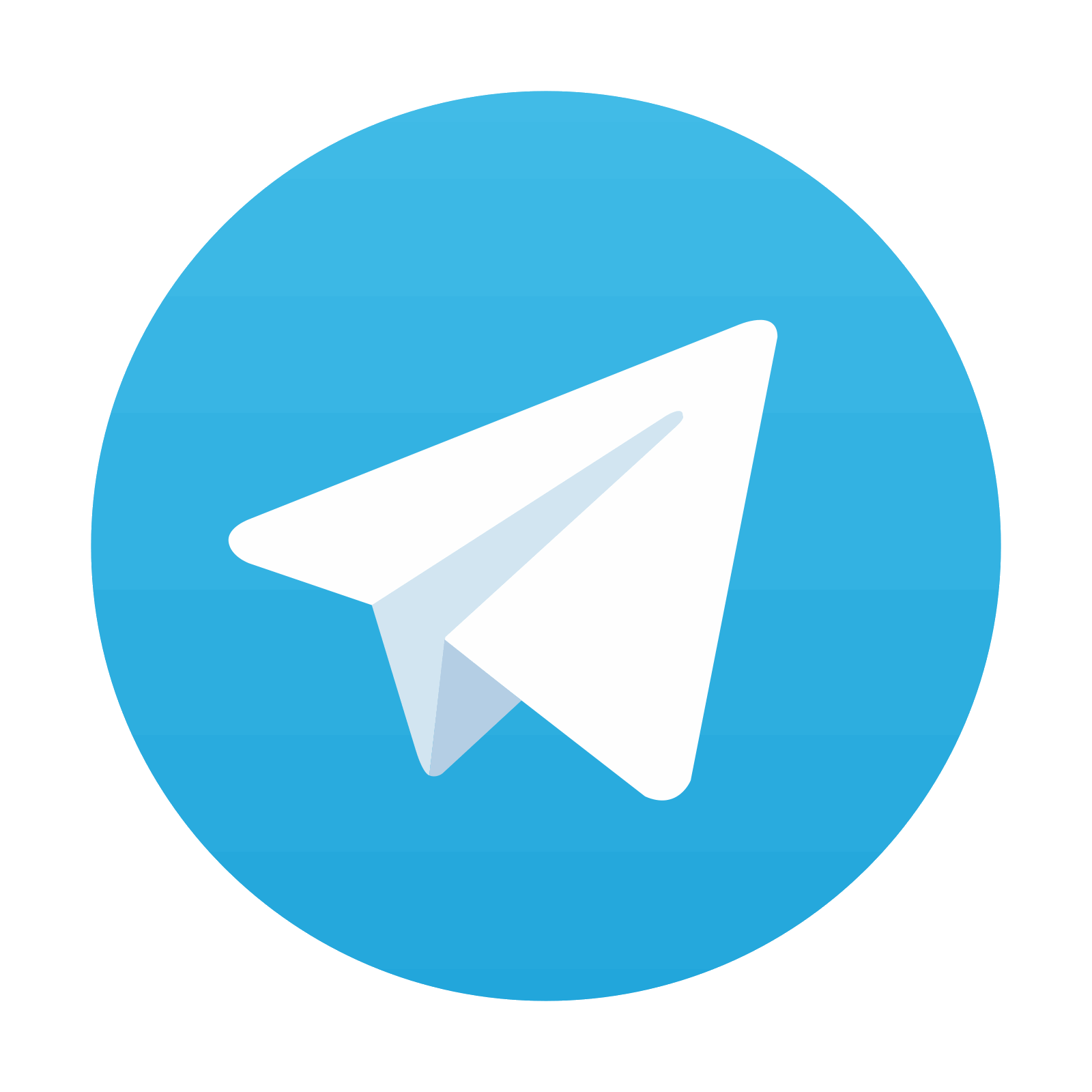
Stay updated, free articles. Join our Telegram channel
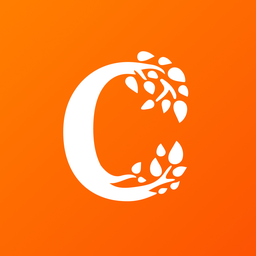
Full access? Get Clinical Tree
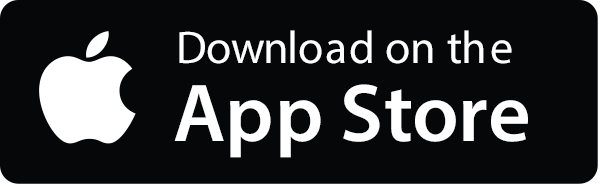
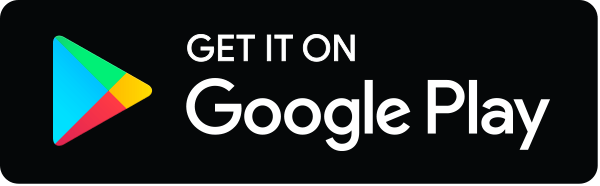