CHAPTER 14 The well-known increase in maximal oxygen uptake ( Peripheral changes due to extended rest include a decrease in capillary density (Poole and Erickson, 2008) and over the long term (>12 weeks), a decrease in mitochondrial density with an associated decrease in oxidative (citric acid cycle) enzymes (Poole and Erickson, 2008). Again, without frequent and regular stress, there is no need for the cardiovascular system to maintain an elevated capacity for the delivery of oxygen to the muscle. Therefore, the increase in muscle capillarization that occurs with training will be lost (Poole and Erickson, 2008). Training adaptations in the muscle itself involve the synthesis of new intercellular structures or enzymes. These positive peripheral changes are more enduring with detraining than are central changes. Mitochondrial density, oxidative enzyme concentration, and myoglobin will diminish with detraining. However, studies have shown that even after 6 months of detraining, up to 50% of the gains made in these parameters remain in place (Rivero and Piercy, 2008). Detraining also results in a decrease in muscle glycogen synthesis and, thus, a decrease in muscle glycogen stores (Rivero and Piercy, 2008). Other detraining changes related to energy or metabolism include a decrease in lipolysis during exercise and a decrease in lactate threshold (OBLA) (Rivero and Piercy, 2008). The increased energy demand of exercise is met, in part, by an improved ability to mobilize and metabolize fat during higher intensities of work. When this demand is absent (i.e., stall rest), this adaptation is diminished and eventually will return to its pretraining capacity (Rivero and Piercy, 2008). Similarly, the training-improved mechanisms responsible for counteracting exercise acidosis will return to their pretraining capacities during detraining. This loss is best illustrated by the occurrence of OBLA at a lower oxygen consumption (milliliters per kilogram per minute [ml/kg/min]) following detraining (Rivero and Piercy, 2008). Skeletal muscle atrophy is an apparent consequence of detraining. Training-induced protein synthesis results in an increase in myofibrils inside the cell. In particular, there is a significant increase in myosin heavy chain proteins (Rivero and Piercy, 2008). Along with this increase is a hypertrophy of the cell itself. With cessation of training, there is an associated loss of myosin heavy chain proteins, and thus, cellular atrophy occurs (Rivero and Piercy, 2008). Detraining and disuse can also alter the strength and integrity of bones, ligaments, and tendons. Although longitudinal data on the effects of detraining on bone in horses are lacking, there is an abundance of information demonstrating that there is a substantial decline in bone mineralization and strength with casting, weightlessness, bedrest deconditioning, and hindlimb suspension. Clinical reports involving horses have suggested diminished bone strength during periods of casting or suspension for the care of animals with severe orthopedic injuries (Hutchins et al. 1987; Nunmaker, 2002). More information is needed to determine if long-term stall housing and lack of activity alter bone, ligament, and tendon strength in the horse. The number of horses over 20 years of age is increasing every year, and many of these animals are still performing various athletic activities (Hintz, 1995; McKeever, 2002; Rich, 1989). As with older humans, the reasons older horses are able to continue to compete include genetics, better general health care, and continual improvements in nutritional management (Hintz, 1995; Malinowski et al., 2002; McKeever, 2002; McKeever and Malinowski, 1997; Ralston and Breuer, 1996; Ralston, 1992; Rich, 1989). Studies of older humans show that overall working capacity decreases with age. The associated declines in aerobic capacity and muscular strength have been attributed, in large part, to the effects of aging on overall physiologic function. However, there is debate in the literature on how much of the age-associated decline in physical performance is due to actual physiologic aging versus disease processes related to inactivity. Research points to the fact that some of the decline in exercise capacity in older humans is related to a general decline in physical activity rather than to physiologic aging (Dempsey and Seals, 1995; Haskell and Phillips, 1995; Holloszy, 1993; Holloszy and Kohrt, 1995). Studies of older humans have shown that both dynamic and resistance exercise training forestalls or even reverses the decline in cardiopulmonary performance and muscular strength (Dempsey and Seals, 1995; Ekelund et al., 1988; Haskell and Phillips, 1995; Holloszy, 1993; Holloszy and Kohrt, 1995; Lakatta, 1995; Raven and Mitchell, 1980; Stamford, 1988). Such results base a strong argument in favor of the preventability of age-related decreases in performance that are rooted in disease or inactivity. Limited data have been published regarding the exercise capacity of the aged horse (Betros et al., 2002; Horohov et al., 1999; Lehnhard et al., 2004; Lehnhard et al., 2001; Malinowski et al., 2002; McKeever, 2002; McKeever et al., 2002; McKeever and Kearns, 2001;; McKeever and Malinowski, 1999; McKeever et al., 1998; McKeever and Malinowski, 1997). Existing studies have focused on establishing a physiologic profile of the older equine, which may or may not have included a single bout of exercise performance. There has been, and continues to be, extensive research to determine the nutritional needs of the older horse (Ralston, 1992; Ralston and Breuer, 1996) however, there have been few studies, to date, to determine physiologic adaptations to training in horses 20 years of age or older. In humans, aging appears to have a significant effect on lung function during exercise (Davies et al., 1988). A review of the literature (Dempsey and Seals, 1995) suggests that there are multiple alterations in pulmonary function that can limit respiratory capacity in older individuals. Studies reporting flow–volume loops suggest that an expiratory flow limitation occurs at lower work intensities in older individuals. Dempsey and Seals (1995) also pointed out the fact that the elastic recoil of the lung is altered with aging, a change reducing expiratory flow rates. Older humans also have a greater dead space that increases the dead space to tidal volume ratio. All together, these age-related changes affect the work of breathing during exertion. Lung hemodynamics are also affected by aging-induced decreases in arteriolar compliance, a detrimental change that may lead to capillary stress failure. If this decrease in compliance should occur in the horse as well, it would have significant implications, since capillary stress fractures are part of the etiology of exercise-induced pulmonary hemorrhage. Despite all of these age-related changes in human lung physiology, Dempsey and Seals (1995) reported that alveolar to arterial gas exchange and pulmonary vascular hemodynamics are only slightly modified. In the horse, there is a void of research regarding the effects of aging by itself on the respiratory response to exercise. It is known that the normal young equine lung is not large enough to handle the demand of high-intensity exercise (Lekeux and Art, 1994). It is also well recognized that factors affecting pulmonary health can have a cumulative effect in the horse. A lifetime of exposure to pathogens and allergens can lead to small airway disease (Lekeux and Art, 1994). Pathologies such as hyper-reactive airway disease, chronic obstructive pulmonary disease (COPD), and exercise-induced pulmonary hemorrhage tend to be more prevalent in older animals (Lekeux and Art, 1994). Such conditions can negatively impact respiratory function during exertion (Lekeux and Art, 1994). However, data are needed to determine the specifics of aging-induced respiratory changes in the horse and their similarity or dissimilarity to humans. Aging has profound effects on the cardiovascular system. In and of itself, the aging process results in a decreased maximal heart rate (HRmax), decreased vascular compliance, changes in baroreceptor sensitivity, and the possibility of concomitant hypertension in such species as rat, dog, and human (Dempsey and Seals, 1995). Functional studies in the horse have demonstrated a similar age-associated decrease in HRmax with a related decrease in In humans, decreases in HRmax with age appear to be caused by several factors, including changes in the number of pacemaker cells in the sinoatrial (SA) node, increases in elastic and collagenous tissues in all parts of the conduction system, and the deposition of adipose tissue around the SA node (Lakatta, 1995). In humans, aging also alters the autonomic tone, with a resulting downregulation of sensitivity to the sympathetic nervous system. This appears to influence the ability to increase HR during exercise (Lakatta, 1995). The horse appears to use autonomic mechanisms in the same way as do humans for the control of HR. Indirect evidence suggests that the horse may also undergo the same aging-induced changes in the neuroendocrine control of cardiovascular function (Goetz and Manohar, 1990; McKeever and Malinowski, 1997; McKeever and Hinchcliff, 1995). A decrease in HR will result in a decrease in cardiac output (Q) unless compensated by an increase in stroke volume (SV) (Q = HR × SV). Data, however, are mixed regarding the effect of age on SV. In unfit older humans, studies have demonstrated a decline in maximal SV, whereas their fit counterparts have maintained, or even increased, this function (Dempsey and Seals, 1995; Ekelund et al., 1988; Haskell and Phillips, 1995; Holloszy and Kohrt, 1995; Holloszy, 1993; Lakatta, 1995; Raven and Mitchell, 1980; Reaven, 1995; Seals, 1993; Seals and Reiling, 1991; Seals et al., 1984; Stamford, 1988). SV is a function of EDV and EF (SV = EDV × EF). A greater filling volume of the left ventricle (EDV) results in a greater myocardial preload (stretch). This greater preload produces a greater myocardial force of contraction, resulting in a greater EF and, thus, a greater SV. Left ventricular hypertrophy (increased EDV) is a well-documented adaptation to endurance training in humans. Studies suggest that older humans are still able to achieve this beneficial training adaptation, compensating, to some extent, for the age-associated decrease in HRmax and, thus, better able to maintain Q. However, in very old individuals, there appears to be both a decrease in HRmax and SV that certainly results in a decline in maximal cardiac output (Dempsey and Seals, 1995; Holloszy and Kohrt, 1995; Lakatta, 1995; Raven and Mitchell, 1980; Stamford, 1988). Until recently, data on the effects of age on central cardiovascular function, cardiac output, HRmax, and SV have been lacking in horses. However, a recent study by Betros et al. (2002) attempted to determine if any of the age-related declines in cardiovascular function were reversible with training. It was found that HRmax (218 versus 213 beats per minute [beats/min]), Since Older horses undergo a decline in Interestingly, when compared with their human counterparts (60 to 80 years of age), the older mare (>20 years) has a tremendous innate aerobic capacity (McKeever and Malinowski, 1997). For example, Besides the ability to accomplish greater workloads, a second benefit of a higher
Age and disuse in athletes: Effects of detraining, spelling, injury, and age
Detraining and disuse
O2max) that occurs with training is reversed relatively quickly when injury or other reasons dictate prolonged rest (Poole and Erickson, 2008). In humans, the cessation of training can result in significant decreases in
O2max within a matter of 2 to 3 weeks. However, in the horse, this decline appears to be slower, and it has been reported that extended stall rest or turnout, following cessation of a 9-month training program, will cause significant decreases in
O2max within 12 weeks, with a much longer period required to return to pretraining levels (Tyler et al., 1996). The increase achieved in cardiac output with training is the first adaptation to reverse itself with detraining (Poole and Erickson, 2008). Maximal end-diastolic volume (EDV), ejection fraction (EF), and, therefore, stroke volume (SV) return to pretraining values within the first 2 weeks of detraining in humans; however, the changes seen in horses appear to take longer, declining between 4 and 12 weeks of deconditioning (N. Kriz et al., 2000). Without the regular occurrence (training) of cardiovascular stress, there is no need for the heart to maintain its left ventricular hypertrophy. Since this hypertrophy is caused by a mere stretching of the walls of the left ventricle and does not involve any protein synthesis of new tissue, the return to pretrained EDV is rapid.
Effect of aging on exercise capacity
Aging-induced changes in respiratory function that may impact exercise
Age-related changes in the cardiovascular response to exercise
O2max (Betros et al., 2002; McKeever, 2002; McKeever and Malinowski, 1997). This overall loss in working capacity is similar to that observed in older humans (Haskell and Phillips, 1995; Holloszy, 1993; Holloszy and Kohrt, 1995; Lakatta, 1995; Raven and Mitchell, 1980; Stamford, 1988).
O2max (116 versus 109 mL/kg/min), maximal oxygen pulse (OPmax ) (0.55 versus 0.52 mL/kg/beat) velocity at HRmax (9.0 versus 9.3 meters per second [m/s]) and velocity at
O2max (8.8 ± 0.2 m/s versus 8.8 ± 0.2 m/s) were similar in young and middle-aged horses (Betros et al., 2002). However, there appeared to be a breakpoint once a horse was over 20 years of age. Old horses had a lower HRmax (193 beats/min),
O2max (95 mL/kg/min), and OPmax (0.43 mL/kg/beat). In addition, the older horses reached their maximal values at lower velocities compared with young and middle-aged horses (Betros et al., 2002). Interestingly, the authors found that training resulted in substantial improvements in
O2max and OPmax but did not alter HRmax in young, middle-aged, or old horses (Betros et al., 2002). First, the results of this study support the occurrence of an age-related decline in HRmax and maximal SV in the horse. This is important for horse owners who use HR to judge the intensity of the work their horses are performing. Second, these results document that training can partially reverse some of the decline in cardiovascular function that occurs in the older horse (Betros et al., 2002).
O2max is the product of the ability to deliver oxygen to the body’s tissues (Q) and their ability to utilize it (a-vO2 difference), the decline in HRmax (and, therefore, maximal cardiac output) most likely contributes to the decline in
O2max (and, therefore, working capacity) seen in older humans and horses. Data are mixed, however, regarding the relative contribution of central versus peripheral factors toward the decline in exercise performance seen in horses, dogs, and humans (Betros et al., 2002; Dempsey and Seals, 1995; Haidet and Parsons, 1991; Holloszy and Hohrt, 1995; Lakatta, 1995; McKeever and Malinowski, 1997; Raven and Mitchell, 1980). In humans and other species, an age-related decline in central cardiovascular function accounts for some of the observed decrease in
O2max. However, decreased muscle mass, alterations in muscle capillary density, and decreased vascular compliance may also limit exercise capacity by limiting blood flow to working muscles (White, 1995). Thus, some of the decline in aerobic capacity is also caused by changes in peripheral mechanisms affecting the ability to utilize oxygen. Unfortunately, these data have been extrapolated from submaximal studies of humans, and the debate whether age-related declines in cardiovascular capacity in humans are predominated by central or peripheral mechanisms continues.
O2max as also seen in healthy older humans. This decline was first documented in horses over 20 years of age by McKeever and Malinowski (1997). More recent studies have shown that the decline in
O2max appears to start at 18 to 19 years of age (Walker et al., 2010) (Figure 14-1). In horses over 20 years of age, a lower
O2max was achieved during an incremental exercise test compared with their younger mature counterparts. Although submaximal oxygen consumption appeared to be similar in young and old horses, the workload needed to reach
O2max was lower in older horses (McKeever and Malinowski, 1997). These observations were similar to well-documented, aging-induced decreases seen in humans (Dempsey and Seals, 1995; Holloszy and Kohrt, 1995; Raven and Mitchell, 1980; Stamford, 1988).
O2max in moderately fit, healthy, postmenopausal women averages 22 mL/kg/min (Holloszy and Kohrt, 1995; Raven and Mitchell, 1980), whereas, young, elite, female athletes typically have capacities ranging from 60 to 80 mL/kg/min (Haskell and Phillips, 1995). Although the average
O2max of 90 mL/kg/min seen in old mares is well below their younger fit equine counterparts (145–200 mL/kg/min), it is still above the level reported in elite human athletes (McKeever and Malinowski, 1997).
O2max is a delay in the need to increase the rate of glycolysis to fuel higher-intensity exercise (McKeever and Malinowski, 1997). Younger horses must work harder to reach their “anaerobic threshold.” This is the point where one observes the onset of blood lactate accumulation (OBLA) conventionally marked by a blood lactate concentration of 4 millimoles per liter (mmol/L) (McKeever and Malinowski, 1997). At this point, there is a curvilinear increase in blood lactate concentration, indicating that lactate production by the working muscles has exceeded lactate utilization throughout the rest of the body (McKeever and Malinowski, 1997). This variable is important, as the velocity to produce a blood lactate concentration of 4 mmol/L (VLA4 ) coincides with changes in several important physiologic processes. Older horses appear to reach VLA4 at both a lower speed and at a lower percentage of their
O2max, which suggests a possible central limitation on the ability to perform work (McKeever and Malinowski, 1997). However, the older mares were also not able to run as long or as hard (Vmax) before reaching fatigue, which suggests a reduction in factors affecting the peripheral mechanisms associated with general exercise tolerance.
Stay updated, free articles. Join our Telegram channel
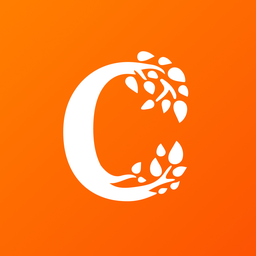
Full access? Get Clinical Tree
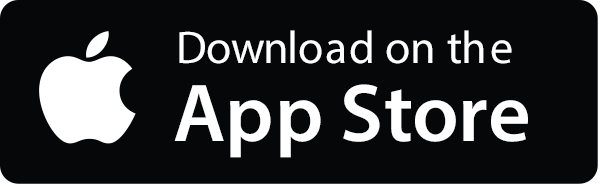
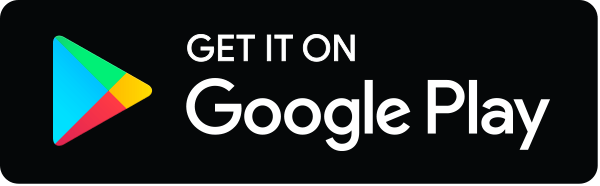