The past 20 years has seen considerable interest in the acid-base state of racehorses at rest. This is in large part due to the practice of horsemen administering alkalinization agents to horses for the purpose of enhancing race performance, i.e. delaying onset of muscle fatigue.1 The reader is referred to the following papers for discussion of ergogenic effects of alkalinizing agents.2 Because of the potential of alkalinizing agents to enhance race performance, regulations have been put in place by all major racing jurisdictions. Most jurisdictions use a threshold for total CO2 (TCO2) of either 36 or 37 mmol/L for horses that have not been given furosemide; the corresponding [HCO3−] thresholds are 34 and 35 mmol/L. Furosemide administration produces a dehydration with attendant changes in plasma ion concentrations, which typically raises TCO2 by ~1.5 mmol/L. Therefore in racing jurisdictions that allow furosemide administration the TCO2 testing threshold can be as high as 39 mmol/L. Racing jurisdictions also contend that all horses having a TCO2 at or above the threshold have been given an alkalinizing agent for the purposes of enhancing exercise performance. Horsemen caring for horses that have been tested at or above the threshold are generally heavily penalized. There is scientific evidence both for and against the use of TCO2 / [HCO3−] testing thresholds, and this has led to considerable debate and legal wrangling. Two main points arise from objective review of the acid-base literature as it pertains to resting horses: 1. Acid-base state and TCO2 are more variable and of a wider range than admitted by racing jurisdictions; a review of the literature shows a large range of natural variability in Standardbred racehorses (Table 39.1). Table 39.1 Acid-base state of race horses in non-race situations Auer et al. 1993:3 TCO2 27–331; 192 horses in stable; Australia Auer et al. 1993:3 TCO2 27–36; 61 horses on raceday, after bicarbonate testing implemented; Australia Kallings, Bondesson and Houghton 1994:4 TCO2 31.4 – 35.2; 6 trotters, Sweden Short 1992:5 [HCO3−] 27–37; 205 trotters and pacers; New Zealand; ‘data from a large group of horses under race-day conditions in which there is reasonable assurance that no bicarbonate was given.’ Short 1992:6 [HCO3−] 25–33; 95 racehorses; Australia Soma et al. 1996:7 [HCO3−] 24.6–33.6; 30 racehorses, Pocono Downs, non-race day Soma et al. 1996:7 [HCO3−] 28.1–34.6; 30 racehorses, Meadows, non-race day Frey et al. 1995:8 [HCO3−] 33–36, mean = 34.5; 12 racehorses in controlled research trial; USA. Therefore TCO2 in 2 of these 12 horses would be between 37 and 38 Waller and Lindinger 2005:9 TCO2 of 30–39, mean 35; 13 Standardbred racehorses on training days at rest, prior to exercise and after morning feeding Nostell et al. 2006:10 TCO2 = 33.2 ± 1.8 (mean ± SE; n = 5) Cohen et al. 2006:11 TCO2 = 31; range 24–37.5; 2,439 Thoroughbred racehorses in California Waller et al. 2010:12 TCO2 of 33.1 ± 2.9 mmol/L; range 25.66–42.9; 211 horses in race training 2. Many factors can contribute to elevated TCO2 in horses that have not been administered alkalinizing agents. From these peer-reviewed studies it can be concluded that plasma [HCO3−] and TCO2 have a wide range of ‘normal’ values within racing populations of Standardbred and Thoroughbred horses. Moderate to high intensity muscular exercise results in acidification of muscles and blood. The acidification primarily results from the generation of protons (H+) within contracting skeletal muscle. The protons are of the product of biochemical and physicochemical reactions associated with increased rates of anaerobic energy production that result in intracellular lactate accumulation, intracellular K+ depletion and increased CO2 production.13 The resultant large and rapid efflux of acid equivalents from contracting muscle produces the systemic metabolic acidosis associated with moderate to high intensity exercise. In the exercising horse, whole-body acid-base balance is dependent on the integrated responses of the muscular, respiratory, vascular, hepatic, cutaneous and renal systems. The muscular system, in addition to providing the locomotory force requirement for activity, generates considerable amounts of acid equivalents, resulting in acidification of the intracellular and extracellular fluid compartments. Non-contracting skeletal muscle also provides the largest tissue mass within the body for the removal of lactate and acid equivalents during high intensity exercise and the initial recovery period. The respiratory system plays a key role in eliminating acid equivalents as CO2 at the lung in addition to extracting the oxygen needed to fuel aerobic cellular metabolism. The vascular system plays an integral role in the transport and distribution of acid and base equivalents throughout the body – this system provides for the ‘buffering’ of the acid-base disturbance by distributing acid equivalents from acid-generation sites (contracting skeletal muscle) to other sites (non-contracting skeletal muscle and other tissues).13 Within the vascular system itself, bicarbonate, plasma proteins and hemoglobin within red blood cells are also involved in the transport and temporary storage (buffering) of acid equivalents. The hepatic system is a major tissue mass involved in the removal of lactate from the vascular system, thereby removing acid equivalents from the circulation. The cutaneous system is heavily involved in the production and secretion of sweat to the surface of the skin during and immediately following moderate to high intensity exercise. Sweat contains large amounts of Na+, K+ and Cl− and different rates of excretion of each ion affects acid-base state of blood leaving the skin.14 The kidneys are capable of excreting H+ and lactate at greatly elevated rates during recovery from high intensity exercise, aiding in the process of recovery from the acidosis of exercise. Why is acid-base balance important? A detailed analysis of acid-base balance can provide a complete biochemical and physicochemical description of the state of the organism, or of individual organs and tissues within the body. Furthermore, severe acid-base disturbances are often associated with high intensity exercise, prolonged duration exercise and with many pathologies. Therefore an understanding of the origins of acid-base disturbance is of interest to both basic and clinical physiologists. Within the context of the present chapter, exercise physiologists remain keenly interested in acid-base balance because of a close association between acidification and muscle fatigue.13 The content of this chapter is primarily directed to moderate to high intensity exercise because exercise at these intensities produces a significant acid-base disturbance, while exercise at low intensities does not (unless markedly prolonged with underlying dehydration and metabolic abnormalities). For detailed reviews of skeletal muscle acid-base balance during exercise the reader is referred to Hultman and Sahlin15 and Lindinger and Heigenhauser.13 Treatments of plasma acid-base status have been provided by Constable,16,17 Johnson et al.18 and Lindinger and Heigenhauser.13 There are also a number of brief reviews on the effects of exercise on acid-base status in horses.16,17,19,20 There are also a number of clinical primers on assessing and treating acid-base disturbances.17,21–23 There is no question that high intensity muscle contraction results in intracellular acidification13,39,40 that generates an extracellular, systemic acidosis in the whole organism that can be very pronounced and long lasting.9 It is also clear that intracellular acidosis and fatigue are associative during high intensity exercise, with mounting evidence that increased [H+] reduces the calcium sensitivity of the contractile proteins.41 Furthermore, acidosis imposed prior to the period of high intensity exercise results in an earlier onset and more pronounced skeletal muscle fatigue.42–44 Intracellular acidosis may, however, only exert these effects during high intensity muscle contraction. Recent evidence has shown that the contributions of intracellular acidosis to fatigue process have yet to be fully understood (Table 39.2). Westerblad and colleagues33 have suggested that increased intracellular concentrations of inorganic phosphate may be a more important contributor to muscle fatigue than the increase in [H+]. Pedersen et al.37,38 have shown that intracellular acidosis can contribute to sustaining muscle function during contractile activity by improving membrane excitability, an effect that appears to involve alterations of sarcolemmal chloride conductivity.45,46 Table 39.2 Effects of increased intramuscular [H+] and functional consequence(s) in muscle Skeletal muscle fatigue is also associated with an increased interstitial [K+] as a result of rapid rates of K+ loss through sarcolemmal K+ channels during the recovery phase of action potentials.47,48 This increase in interstitial [K+] results in a marked depolarization of the sarcolemma and decreased contractile force.48,49 In contrast to the dogma that we have long been taught, the loss in both sarcolemmal excitability and tetanic force resulting from elevated interstitial [K+] (8–12 mEq/L) was actually reversed when intracellular acidosis (either 20 mmol/L lactic acid or increased CO2) was imposed.37,50,51 While these muscles were only stimulated to perform one contraction every 10 min, this allowed a separation between the fatigue associated with repetitive contraction versus that associated with sarcolemmal depolarization and intracellular acidification. As summarized by Fitts52 and Allen,40 increased [H+] does contribute to decreased force production during high intensity muscle contraction (see Fig. 39.1), and there is reasonably good evidence that these effects occur at the level of: (i) impaired Ca2+ binding to troponin C, which therefore impairs the ability of actin to form cross-bridges with myosin; (ii) slowing SR Ca2+-ATPase activity; (iii) increasing the leak of Ca2+ from the SR; (iv) and at key sites of biochemical control within glycogenolysis (decreased glycogen phosphorylase a activity) and glycolysis (decreased phosphofructokinase activity. The latter study also demonstrated an increased reliance on fat metabolism to meet the energy demands of contracting muscle during exercise in humans made acidotic by ingestion of 0.3 g/kg ammonium chloride.24 In traditional terms, many of us remember being taught that acid-base balance is represented by the relationships among PCO2, pH and the HCO3− in blood plasma. While this is true, using only these three variables provides for only a very limited understanding of the factors that contribute to acid-base imbalances. The approach taken within this chapter is to use a comprehensive, physicochemical approach to identify the causes or origins of acid-base disturbances during exercise, and to discuss how the disturbance is resolved during recovery from exercise.13 Several key papers have used this approach in horses.9,12,53–58 The physicochemical approach presented here was detailed by Peter Stewart.59–62 The foundation for this approach lies on the work of van Slyke and co-workers,63,64 and builds on the work of many others including Hastings, Dill, Henderson and Siggaard-Anderson.13 The advantages and disadvantages of the physicochemical approach are listed in Table 39.3. The development and widespread use of ion selective electrodes and combination blood gas–electrolyte analyzers has greatly simplified the process of obtaining the necessary measurements with the accuracy needed to perform detailed assessments of acid-base balance.13 The advantages of this approach lie in the ability to quantitatively determine the physical and chemical origins of acid-base disturbances. This is therefore a very powerful approach and an important step towards understanding acid-base physiology and pathophysiology. This approach provides an essential foundation for the effective treatment of pathological acid-base disorders. Table 39.3 Disadvantages and advantages of the physicochemical approach 1. A dissociated proton molecule (H+) is only in physical existence for a fleeting instance of time, approximately 10−5 second. The proton is highly reactive, associating briefly with negative charges on proteins, −OH molecules, HCO3− molecules and amino acids to name a few. The proton is therefore very unlike inorganic electrolytes such as Na+, K+ and Cl− that are relatively unreactive. 2. Protons are a main constituent of water, the most prevalent molecule within the body. Water thus provides an almost limitless source of H+ for biochemical and physicochemical reactions. Protons are part of the solvent that comprises the milieu of the body. It is because of its ability to so rapidly dissociate and re-associate H+ and −OH that water is the ‘universal’ solute. 3. Because of these physical attributes of protons and water, it is physically impossible to add protons to a physiological solution without adding water. Take hydrochloric acid (HCl) as an example. HCl exists only in aqueous form and is characterized by a very high concentration of Cl− and H+ in solution. The H+ is an integral part of the aqueous system. It will be described below that it is the strong acid anion Cl− that makes this solution so acidic. The strong acid anion Cl− can be neutralized by the addition of an equivalent amount of the strong base cation Na+ to the solution, but without an accompanying acid anion such as Cl−, HCO3−, of H2PO4−. Thus NaOH would be added – the strong anions Cl− and Na+ remain fully dissociated in solution while there occurs a rapid reaction between H+ and −OH that decreases [H+]. The resultant solution is saline at neutral pH. The terms strong acid anion and strong base cation were introduced in the preceding section and they will be defined here. The term ‘strong’ refers to the fact that the ion will be fully, or nearly so, dissociated in aqueous solutions (Fig. 39.2). Most of the inorganic ions are ‘strong’ and hence nearly fully dissociated within the body fluids (Table 39.4). Some organic ions are also strong, such as lactate− (acid dissociation constant of 3.9) and phosphocreatine2− (PCr2−; acid dissociation constant of 4.5). Anions possess negative charge while cations possess positive charge. An anion is an acid by definition because the addition of that strong anion, in the absence of an accompanying strong base, will result in acidification of the solution. Using the example of HCl above, the addition of HCl to plasma will result in acidification. Similarly, the addition of HLactate will also result in acidification. In contrast, the addition of the strong base Na+ in the absence of accompanying strong anion (as NaHCO3−) will result in alkalinization. The values for the key variables used in the physicochemical assessment of acid-base balance, for resting horses, are provided in Table 39.5. Table 39.4 A summary of acid-base terminology Table 39.5 Physiologically important acid-base variables, and their concentrations, in arterial plasma and skeletal muscle of horses at rest na = not applicable. aSahlin et al.67 – human muscle. bHultman and Sahlin15 – human muscle. Within plasma and the extracellular fluid compartment, the [SID] may be calculated as: In some treatments of acid-base balance using the physicochemical approach, [lactate−] is also ignored. However [lactate−] cannot be ignored in the exercising and recovering animal. The term ‘weak’ refers to those anion acids and cation bases that are not fully dissociated in solution. Thus when sodium phosphate (Na2HPO4) is added to an aqueous solution, two Na+ are added and fully dissociates and a weak anion HPO42− is added. In contrast to Na+, the HPO42− cannot achieve full dissociation due to reactions of the molecule with H+ within the solution. Thus the HPO42− is also partially and instantaneously transformed into H3PO4, H2PO4− and PO43− (Fig. 39.2). This physical attribute of phosphate is what makes phosphates, and many other weak acid anions such as bicarbonate and albumin, good proton ‘buffers’. The predominant weak acid anion in plasma and ECF is albumin, while the predominant weak acid anions within skeletal muscle cells are the histidine residues on proteins. The main weak acids and bases within the extracellular fluid compartment are albumin, globulin, phosphate and bicarbonate. Bicarbonate, however, is part of the CO2 system and thus is not used in the calculation, or estimation, of [Atot]. As for the strong ions, the weak ions also directly affect the concentrations of H+ and HCO3− in solution. Within skeletal muscle it is primarily the histidine moieties on proteins that contribute to [Atot], with creatine, Pi, ATP and other molecules also contributing.68 While it is theoretically possible to measure the concentration of weak acids and bases in both extracellular and intracellular fluid compartments, this tends to be prohibitive and appears not to be necessary to be able to effectively estimate acid-base state. Rather, an effective [Atot] and apparent dissociation constant K’a have been determined in equine plasma and rat skeletal muscle (Table 39.6). A value for [Atot] has not been determined in equine or human skeletal muscle. Muscle [Atot] is equivalent to the non-bicarbonate proton buffering capacity of adult rat plantaris muscle,68–70 and is similar to that of human vastus lateralis.15,71,72 When rat plantaris values for [Atot] and K’a were applied to human muscle, reasonably good data were generated.68 Equine muscle, compared to human muscle, has a much greater non-bicarbonate proton buffering capacity: 43 mEq.kg dry muscle−1.pH−1 in trained humans, vs 58 and 93 mEq.kg−1.pH−1 in untrained and trained equine skeletal muscle.73 Assuming proportionality with rat hindlimb skeletal muscle (buffer capacity of ~40 mEq.kg−1.pH−1 = [Atot] of ~140 mmol/L68), this translates to an [Atot] of ~315 mmol/L in trained equine muscle. Table 39.6 Values of the constants used within the acid-base equations The concentration of CO2 is the third independent variable of acid-base balance. Carbon dioxide is effectively a strong acid, and because it is a major end-product of cellular respiration is often referred to as a respiratory acid. Also, the primary means for eliminating excess CO2 from the body is through the respiratory system.13,18 With this background, the following five mass action equations and one equation expressing electrical neutrality of solutions describe the physicochemical characteristics of any aqueous, physiological solution:60–62 Weak electrolyte system: Electrical neutrality: When considering the acid-base changes that occur in blood during exercise, it is important to have an understanding of the changes that occur within skeletal muscle because that tissue forms 40–60% of the mass of the horse.75 Contracting skeletal muscle generates the disturbance76,77 and non-contracting cells are capable of ameliorating the disturbance.78,79 The role of non-contracting muscle may be small to negligible in horses performing moderate to high intensity exercise because most skeletal muscles are used for locomotion and maintenance of posture. That is in contrast to bipedal humans where many activities require leg muscles and leave many other muscles relatively inactive. This section will thus focus on the time course and magnitude of changes that occur within contracting skeletal muscle, primarily gluteus medius, during moderate to high intensity exercise. The acid-base changes that occur within contracting skeletal muscle and in blood during exercise are the results of the biochemical (metabolic) and physicochemical reactions that occur within contracting muscle cells.13 The onset of muscular contraction sets into motion a series of biochemical events that result in stimulation and inhibition of numerous metabolic pathways. Those pathways within the aerobic energy systems are relatively slow to increase, while those of the anaerobic pathways (ATP utilization, phosphocreatine degradation, glycolysis) increase rapidly. Thus the onset of exercise (rest to work transition) may be associated with muscular acidification for reasons described below. Similarly, transitions from low to high work rates, as well as exercise at moderate to high intensities, result in increased rates of anaerobic metabolism. Full activation of aerobic pathways may be achieved within minutes of the onset of exercise, but until this is achieved anaerobic pathways continue to supply ATP. Activation of aerobic metabolism results in increased mitochondrial respiration with CO2 production – while this CO2 is acidic, its rate of production and removal from the cell can easily be matched by CO2 elimination rates at the lung. Therefore aerobic CO2 production can be ignored in most discussions of the acid-base changes of exercise. Skeletal muscle is composed of different fiber types, some of which produce acid equivalents at high rates (the anerobic, fast twitch, glycolytic fibers) while others do not (the aerobic, slow twitch, oxidative fibers). Fiber types continue to be classified on the basis of their twitch characteristics, oxidative / glycolytic capacities and on their myosin heavy chain composition.80 The acid-base changes that occur reflect the fiber type composition of the contracting muscles, and may reflect breed differences and type of activity performed. This is tempered by the high degree of variability in muscle fiber type proportions in most horse breeds.81,82 Within individual muscle groups, such as the well-studied gluteus medius of equids, skeletal muscle fibers of different composition are in close proximity and form integrated functional units that are selectively recruited by appropriate motor units depending on the locomotory requirements of the animal.83 Muscle fibers with high oxidative capacity that have low glycoytic capacity, slow contractile properties with low myosin ATPase activity, are fatigue resistant and primarily function in the maintenance of posture (Table 39.7). These slow twitch oxidative fibers have the ability to oxidize all the pyruvate generated from glycolysis and, during exercise, they have the ability to take up and oxidize lactate released into the interstitium from nearby glycolytic muscle fibers – the intramuscular lactate shuttle.84
Acid-base physiology at rest, during exercise and in response to training
Acid-base balance in resting horses
Overview of acid-base balance
Acidosis and skeletal muscle fatigue
Assessment of acid-base balance and factors that affect acid-base regulation
Physicochemical determinants of acid-base balance
Strong ions and strong ion difference
VARIABLES
PLASMA
SKELETAL MUSCLE
Dependent variables
‘normal’ value
normal range
‘normal’ value
normal range
[H+] nEq/L
40
33–45
100
71–126
pH
7.40
7.35–7.48
7.0a,b
6.90–7.15a,b
[HCO3−] mEq/L
28
22–34
10a
8–12a
Independent variables
PCO2 mmHg
40
35–45
[total CO2] mmol/L
30
23–36
10a
8–12a
[SID] mEq/L
40
37–43
110c
60–130c
Strong ions
[Na+] mEq/L
140
132–146
15
8–30
[K+] mEq/L
3.7
2.7–4.7
140
110–165
[Ca2+] mEq/L
2.5
2–3
0.4
0.2–1.0
[Mg2+] mEq/L
1.0
0.5–2.0
5
3–7
[Cl−] mEq/L
105
99–109
10
5–25
[lactate−] mEq/L
1.0
0.5–1.5
1.5
1.0–2.0
[PCr2−] mEq/L
na
na
15
12–18
[SO42−] mEq/L
0.5
0.3–0.7
–
–
Weak ions
[Atot] mEq/L
12
11–13
140
120–170
[plasma protein] g/dl
5.5
5.0–6.0
na
na
[albumin] g/dl
na
na
[globulins] g/dl
na
na
[HPO42−] + [H2PO4−] mmol/L
2.7
2.0–3.5
8
7–9
carnosine mmol/L
na
na
6b
3–9b
Protein histidine concentration mmol/L
–
–
46b
30–60b
Weak acids and bases, and [Atot]
PARAMETER
CONSTANT
REFERENCE
KA – plasma
2.11 or 2.12 × 10−7 Eq/L
Constable 1997;17 Staempfli et al. 199974
1.74 × 10−7 Eq/L
Waller et al. 201012
KA – resting muscle
1.64 × 10−7 Eq/L
Lindinger 199568
KA – exercised muscle
1.98 × 10−7 Eq/L
Lindinger 199568
K3
6.0 × 10−11 Eq/L
Stewart 198362
KC
2.46 × 10−11 (Eq/L)2/mmHg
Stewart 198362
K’w
4.4 × 10−14 (Eq/L)2
Stewart 198362
The carbon dioxide system
Solving equations to determine acid-base balance
Contracting skeletal muscle: proton generating and removing reactions
Muscle characteristics and acid-base
Stay updated, free articles. Join our Telegram channel
Full access? Get Clinical Tree
Acid-base physiology at rest, during exercise and in response to training
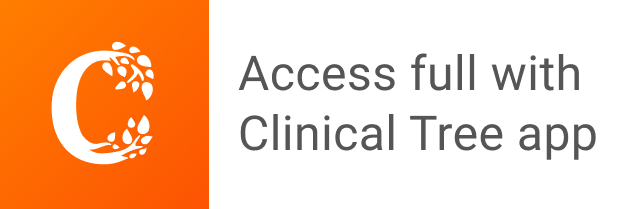