Luc L. Mertens and Andreea Dragulescu The Hospital for Sick Children; University of Toronto, Toronto, ON, Canada Assessment of right ventricular (RV) function is an important topic in congenital and pediatric heart disease, as the RV is affected by different congenital structural defects as well as in patients with pulmonary hypertension [1–3]. Structural defects are often associated with abnormal pressure and/or volume loading of the RV. Additionally, abnormalities in underlying RV myocardial development and structure further make the RV more vulnerable to development of dysfunction and failure. Cardiac surgical interventions with the effect of cardiopulmonary bypass, surgical incisions on the right atrium and RV, and postoperative adhesions of the RV anterior wall to the chest wall further influence the development of RV dysfunction and failure. Recent insights from embryology and molecular biology have demonstrated that the RV myocardium has a different embryologic origin from the left ventricular (LV) myocardium as RV myocardial cells migrate from the secondary heart field [4]. Also the molecular responses to abnormal loading conditions have been described to differ between the two ventricles. This explains why the RV response to pharmacologic treatments such as beta‐blockers and angiotensin‐converting enzyme inhibitors is different from the LV response. This is a developing field of research but it has become clear that monitoring RV function is crucial in different congenital heart defects. RV dysfunction is an important determinant of outcome in patients with tetralogy of Fallot after repair, transposition of the great arteries after atrial switch with a systemic RV, congenitally corrected transposition, hypoplastic left heart syndrome and other forms of single RVs, and pulmonary hypertension. Although MRI techniques are considered the clinical reference technique for quantification of RV size and function, echocardiography will always remain the first‐line imaging technique as it is widely available, can be performed at the bedside, and is associated with lower cost compared to MRI [5]. Echocardiographic quantification of RV function is, however, challenging, and assessment of RV function is often limited to subjective visual evaluation (“eyeballing”). Recent guidelines have stressed the importance of clinical utilization of quantitative techniques that provide more objective measures of RV function [6,7]. For the LV, ejection fraction is considered a single parameter that well represents global LV performance, but there is no corresponding generally clinically accepted echocardiographic RV parameter, as RV ejection fraction is difficult to assess. Generally, a combination of parameters can be used to assess RV function during longitudinal follow‐up of patients. The RV is more difficult to evaluate than the LV because of its more complex geometry, its anterior position in the chest, and its different physiology and mechanics. The RV has a complex 3D shape with a triangular appearance in the sagittal plane and a crescent shape in the coronal plane. The RV can be described as a tripartite structure with an inflow part, a trabecular part, and an outflow component. As the RV inflow and outflow are positioned in different planes, they are difficult to image simultaneously by 2D echocardiographic techniques. A full assessment of the three components requires combining different 2D images or a 3D technique. The normal RV is thin‐walled (around 3–5 mm in the adult) and has prominent trabeculations, which complicate wall detection and endocardial definition. In neonates and in patients with tetralogy of Fallot, pulmonary hypertension, and systemic or single RV, the RV walls are thicker and easier to identify. The apical trabeculations form a complex network of muscular channels in which blood enters in diastole and is squeezed out in systole. Thus, the trabecular network functions as a sponge and is important for RV output. The anterior position of the RV in the chest limits echocardiographic visualization due to limited spatial resolution in the near field of the ultrasound beam. Finally, lung interference can further limit echocardiographic windows. This can be especially problematic in patients with chronic lung disease or in the early postoperative ventilated patients with limited echocardiographic windows in whom evaluation of RV function can be important. Following the predominantly longitudinal orientation of the RV fibers, normal RV contraction is characterized by longitudinal motion of the base towards the apex, and less circumferential and rotational motion. Apart from the longitudinal component, RV contraction is characterized by a bellow‐like motion of the free wall towards the septum and bulging of the ventricular septum into the RV cavity. The normal RV pressure–volume loop has a trapezoidal shape with poorly defined isovolumic periods. This is in contrast to the typical rectangular LV pressure–volume loop, which has clearly defined isovolumic contraction and relaxation phases. Right ventricular output is highly sensitive to increased afterload as a relatively mild acute increase in afterload will lead to reduced RV output. Right ventricular concentric hypertrophic response to chronic pressure loading results in a more important contribution of circumferential fiber shortening to RV performance. This has been demonstrated in patients with congenitally corrected transposition and in hypoplastic left heart syndrome. This adaptation will result in circumferential shortening becoming a more important component of RV systolic performance. Due to its higher compliance, the RV is better suited to accommodate volume changes. Chronic volume loading will result in RV eccentric remodeling and RV dilation with often preserved RV function. Chronic volume loading is, however, not well tolerated in patients with associated RV pressure loading such as in systemic RVs and after surgical correction of tetralogy of Fallot. Right ventricular failure is typically characterized by progressive RV dilation and tricuspid regurgitation. As for the LV, assessment of RV function should include assessment of RV dimensions. Typically, progressive reduction in RV contractile function will result in RV dilation as a mechanism to compensate for decreased output. MRI is considered the clinical reference technique for assessment of RV size as it allows obtaining high‐quality volumetric images with relatively good endocardial definition. While MRI techniques are considered the reference method for calculating RV volumes and RV ejection fraction, the measurements have to be well standardized as different measurement techniques have been proposed [8]. Significant variations in volume can result from different image orientation (axial versus coronal) as well as the inclusion or exclusion of RV trabeculations [9]. Particularly at end‐systole, the endocardial borders can be difficult to identify as the trabeculations are merged with the myocardium, especially when RV function is preserved. Echocardiographic measurements of RV size have been recommended by the American Society of Echocardiography and have also been included in the pediatric measurement recommendations [6,7,10]. These include 2D measurements as well as assessment of RV volumes based on 3D echocardiography. To assess RV size, different 2D echocardiographic views should be obtained to allow full coverage of the RV, including its inlet, trabecular, and outlet components. This includes measurements obtained from apical, parasternal long‐axis, short‐axis, and subxiphoid views (Figure 9.1). From the apical RV‐centric view, RV length, tricuspid valve annulus size, RV1 dimension below the tricuspid valve, and RV2 as the mid‐RV transverse size can be measured. From the same view, RV end‐diastolic and end‐systolic areas can be measured and indexed for body surface area (Figure 9.2). From the parasternal long‐axis and the LV short‐axis views, RV end‐diastolic dimensions can be measured. These are cross‐sectional measurements of the RV trabecular part closer to the RV outflow. Normal RV dimensions are available for the adult population, but for children z‐scores are not available for all measurements included in the recommendations. Two‐dimensional measurements are reliable if well standardized and can be used for monitoring changes in RV size during serial follow‐up. When compared to MRI measurements, the echocardiographic measurements tend to underestimate the corresponding MRI values [11]. From the different available 2D measurements, end‐diastolic RV area as measured from the apical RV‐centric view correlates best with RV volumes measured by MRI [12]. The indexed (for body surface area) RV end‐diastolic area is the best 2D approximation for RV volumes, and in tetralogy of Fallot patients can be used to determine which patients could benefit from additional imaging [13]. Based on correlation analysis with MRI images, a formula to predict RV end‐diastolic volume (RVEDVi) in adult tetralogy patients was proposed by the adult congenital group in Toronto: RVEDVi = 11.5 + (7 × indexed RVED area) [12]. When using this method, it is important to obtain an RV‐centric apical four‐chamber view without foreshortening the RV. Typically, RV trabeculations are included in the RV area calculation, as this is the method used by our MRI specialists when measuring RV volumes. In children after tetralogy of Fallot repair, an indexed RVED area of >20 cm2/m2 has been shown to predict an RV volume index of >170 mL/m2. This illustrates that RVED area could be used to preselect tetralogy patients who could benefit from additional MRI measurements in centers where MRI is less readily available and not part of the routine follow‐up. Figure 9.1 Measuring right ventricular (RV) dimensions from the apical RV‐centric view. RV length is measured from the tricuspid annulus to the RV apex. RV1, transverse dimension of the RV just below the tricuspid valve; RV2, transverse RV dimension at mid‐RV level; TV ann, tricuspid valve annulus. Figure 9.2 Measurement of right ventricular (RV) end‐diastolic (d) and end‐systolic (s) area. Based on these measurements, RV fractional area change can be calculated. In this patient after tetralogy of Fallot repair, fractional area change was 39%, which is within normal range (>35%). Based on 2D measurements, RV volumes can be calculated using complex geometric formulae, but none of these accurately predict RV volumes [14]. Therefore, this approach has largely been abandoned because volumetric models have readily become available. A 2D‐based 3D reconstruction method to calculate RV volumes is based on obtaining 2D images of the RV that are localized in 3D space based on spatial magnetic tracking of the probe position while obtaining 2D images [15,16]. On the 2D images, anatomic landmarks (points) are marked and the 3D volume is reconstructed using a catalogue of reference RV shapes (Figure 9.3). This method is highly accurate as the calculated RV volumes correlate well with MRI‐based volumetric measurements [17]. However the need for a magnetic tracking device and dedicated equipment limits its clinical use. Three‐dimensional echocardiography allows full volumetric data acquisition and subsequent off‐line analysis of RV volumes using dedicated software (Figure 9.4). Different software is available for analyzing RV volumes and has become more automated, allowing this technology to be implemented in clinical practice [18,19]. Despite technological advances, 3D echocardiography for assessing RV volumes and function still has problems that limit its clinical implementation. First, the clinical feasibility of the method is influenced by the image quality of the 3D datasets. Especially when the RV is significantly dilated, it can be challenging to include the entire RV volume in the 3D volumetric acquisition. The anterior RV wall can be particularly challenging to include [20]. Second, the frame rates of the volumetric acquisitions, especially when the RV is enlarged, tend to be low relative to the heart rate which can make it difficult to identify end‐systole and end‐diastole because of limited temporal resolution. In children with higher heart rates, this can result in the underestimation of end‐diastolic volume and overestimation of the end‐systolic volume, affecting the calculated ejection fraction. Finally, high‐frequency 3D probes are not readily available, further limiting spatial resolution in smaller children. Typically, 3D‐based echocardiographic RV volumes are 15–34% lower when compared to MRI‐based RV volumes. This discrepancy is related to difficulties in echocardiographic acquisition (with the RV not completely included in the datasets) and in RV endocardial border detection and definition. Additionally, intra‐ and interobserver variability of 3D volumetric measurements is between 10 and 20%, which needs to be taken into consideration for the interpretation of serial measurements [21,22]. While improvements in RV volumetric acquisition and more automated analysis based on artificial intelligence methods are to be expected in the future, the current technology is challenging to apply in the clinical environment [23]. As for the LV, the most commonly used quantitative methods to assess RV function are based on measuring dimension changes (Table 9.1). Due to its complex geometry and longitudinal contraction pattern, simple 1D or 2D techniques, such as shortening fraction and the biplane Simpson’s or area‐length methods of ejection fraction, that rely on geometric assumptions are not applicable to the RV. Calculation of fractional area change (FAC) from the apical RV‐centric view is a valid alternative. This method measures RV end‐diastolic (RVEDA) and end‐systolic (RVESA) areas from the apical RV‐centric view (Figure 9.2). Figure 9.3 Two‐dimensional‐based 3D reconstruction of right ventricle (RV) volumes. This method uses 2D images that are obtained with a magnetic tracker attached to the probe. On the 2D images, anatomic landmarks are identified (tricuspid annulus, pulmonary valve, septum, and RV endocardium), and the RV volumes are reconstructed based on an online database of RV shapes. Figure 9.4 Three‐dimensional echocardiography can be used for measuring right ventricle (RV) volumes using dedicated analysis software. Table 9.1 Right ventricular (RV) systolic function derived from dimensional changes 2DE, two‐dimensional echocardiography; 3DE, three‐dimensional echocardiography; TAPSE, tricuspid annular plane systolic excursion. A normal FAC value is >35%. This method requires a good‐quality apical view and we trace the RV endocardium at the base of the trabeculations. Feasibility is dependent on the quality of the echocardiographic windows, but FAC measurement is feasible in most pediatric and adult patients. The interobserver variability of the FAC measurement seems to be mainly related to the difficulty of measuring RVESA as the endocardial border may be difficult to define at end‐systole. Interobserver variability can be considerable and good standardization of the methodology is required if FAC is used for clinical follow‐up. It is important to realize that FAC only measures dimensional changes in the inflow and trabecular portions of the RV, which may result in overestimation of global RV systolic function in the presence of RV outflow tract dysfunction as may occur in postoperative tetralogy of Fallot patients. In patients with global RV dysfunction, FAC correlates well with RV ejection fraction (EF) and is a valuable substitute measure for RV EF [24]. In the presence of regional dysfunction, RV EF by 3D methods is the preferred method but may not always be obtainable. As longitudinal shortening is the predominant vector determining RV function, assessment of longitudinal function is an essential component of RV functional assessment. The easiest method for assessment of longitudinal function is measurement of the tricuspid annular plane systolic excursion (TAPSE). This is obtained by placing an M‐mode cursor through the tricuspid lateral annulus (Figure 9.5). We use color Doppler M‐mode to define the onset of systole and the point of maximal systolic excursion. TAPSE measures the distance the RV annulus travels during systole, which is normally >1.8 cm in adults. Because TAPSE is dependent on cardiac size, it should be corrected for age or body size. Age‐dependent graphs of normal values for TAPSE are available [25]. TAPSE has been found to correlate well with RV EF and FAC. It is, however, a regional parameter measuring longitudinal shortening only in the RV lateral free wall and does not represent global RV dysfunction in case of regional changes. By definition, patients after tetralogy of Fallot repair who underwent RV outflow tract patch repair have regional dysfunction in the RV outlet. In these patients TAPSE has been found to correlate only weakly with global RVEF [26,27]. Apart from changes in the RV outflow tract, additional regional differences in RV function in patients after tetralogy of Fallot repair can explain the poor correlation between a basal functional parameter such as TAPSE and global EF, as apical function seems more significantly reduced in postoperative patients [28]. TAPSE is, however, easy to measure and, when well standardized, it is highly reproducible. In postoperative patients TAPSE can be reduced while FAC is preserved. This is likely related to adhesions of the RV anterior wall to the chest wall which can influence the excursion of the tricuspid annulus. Additionally, TAPSE is influenced by LV longitudinal shortening. This can explain why in patients with RV dysfunction TAPSE can remain within normal range in patients with preserved LV function, as the contraction of the LV lateral wall pulls over the RV lateral wall with downward motion of the tricuspid annulus. Finally, the presence of tricuspid regurgitation can influence TAPSE and result in increased annular motion. Serial measurements of TAPSE may be useful in clinical follow‐up, especially in patients with pulmonary hypertension. Hauck et al. demonstrated that in young patients with pulmonary hypertension TAPSE is preserved except in patients after surgical repair for congenital heart disease [29]. When TAPSE decreases over time in pulmonary hypertension patients, it is considered a poor prognostic sign [30]. Figure 9.5 Tricuspid annular plane systolic excursion (TAPSE). An M‐mode cursor is placed through the tricuspid annulus aligned with the longitudinal motion of the annulus during systole. The excursion of the annulus during systole is measured. We use color tissue Doppler to help with the timing of the measurements. Table 9.2 Blood pool and tissue Doppler indices of right ventricular function dP/dt, ratio of change in pressure over change in time; EF, ejection fraction; PVR, pulmonary vascular resistance; RV, right ventricular; TDI, tissue Doppler imaging; TR, tricuspid regurgitation. RV longitudinal motion can also be assessed by tissue Doppler and RV longitudinal strain assessment as discussed in the following sections. Different Doppler‐based methods can be used to assess RV function (Table 9.2). As for the LV, dP/dt could be measured from a tricuspid regurgitation jet, but pressure changes in the RV make dP/dt more difficult to measure. The myocardial performance index (MPI) is defined as a combined index of systolic and diastolic function. It is based on measurement of the sum of the isovolumetric contraction and relaxation times divided by the ejection time. It is measured by obtaining a spectral Doppler tricuspid inflow tracing and a pulmonary valve tracing. Typically, the time from tricuspid valve opening to tricuspid valve closure (a) and the ejection time (b) is measured from those traces. The MPI is calculated as (a – b)/b [31,32]. Because RV physiology is characterized by very short or even absent isovolumic events (especially isovolumic relaxation), the use of isovolumic time intervals to assess RV function is not entirely physiological. Nevertheless the MPI has been applied to the RV. Due to RV geometry these Doppler signals cannot be measured simultaneously, which increases measurement variability when compared to MPI measurements obtained in the LV [33]. When using blood pool data, the upper normal limit is less than 0.4. In the presence of pressure loading and RV dysfunction, the isovolumic time intervals lengthen and the ejection time shortens, resulting in a prolonged MPI. MPI is highly sensitive to changes in loading conditions, which again limits its use in clinical practice. As for the LV, an abnormal MPI can reflect changes in either systolic or diastolic function (or both) and in loading conditions. MPI derived from tissue Doppler was found to correlate well with pulmonary vascular pressures and resistance in pulmonary arterial hypertension (PAH) before and after surgery for thromboembolic pulmonary vascular disease [34]
CHAPTER 9
Right Ventricular Function
Introduction
Echocardiographic assessment of RV size
Right ventricular systolic function parameters
Right ventricular functional parameters from dimension changes
Dimensional changes
Strengths
Weaknesses
Fractional area change (FAC)
Correlates with RV EF, especially when there is global dysfunction
Geometry dependent – does not include the RV outflow tract
Variability due to difficulty of tracing RV endocardial border
Load dependent (preload + afterload)
Ejection fraction (EF) by 3DE
More accurate than 2DE methods to assess RV EF
Reliance on good‐quality 3D datasets
Low frame rates
Underestimation of RV volumes by 10–30%
Load dependent (preload and afterload)
TAPSE by M‐mode
Correlates with RV FAC and EF if no regional dysfunction
Easy and reproducible
Regional basal parameter
Load dependent
Influenced by valve regurgitation
Influenced by LV function
Blood pool and tissue Doppler indices
Strengths
Weaknesses
dP/dt
Geometry independent
Afterload independent
Preload dependent
Clear continuous‐wave Doppler tracing required
Very short time interval limits reproducibility
Myocardial performance index (MPI) by pulsed‐wave and TDI
Geometry independent
TDI‐derived MPI correlates with PVR
Correlates with RV EF
Tricuspid inflow and pulmonary artery Doppler velocities not measured simultaneously
Load dependent (preload and afterload)
Myocardial acceleration during isovolumic contraction (IVA)
Geometry independent
Relatively afterload independent
Heart rate dependence allows for study of force–frequency relationship
Influenced by preload, especially when filling pressures are increased
Systolic‐to‐diastolic duration ratio (S/D ratio)
Geometry independent
Heart rate dependent
Dependent on reliable TR signal
Blood pool Doppler indices for right ventricular function
Stay updated, free articles. Join our Telegram channel
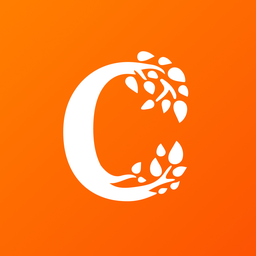
Full access? Get Clinical Tree
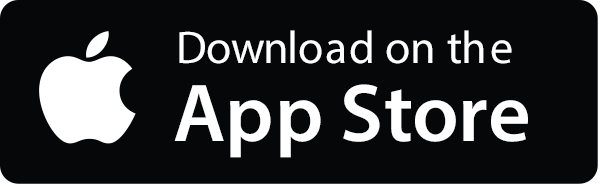
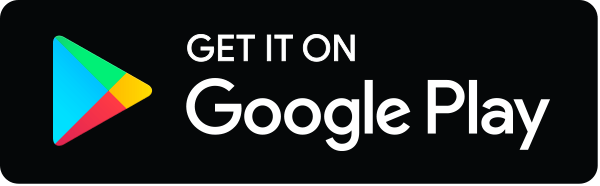