Peter C. Frommelt Medical College of Wisconsin, Milwaukee, WI, USA Echocardiography is the primary tool in the diagnosis and assessment of pediatric heart disease, including abnormalities of ventricular function. The initial focus when assessing myocardial function has traditionally centered on systolic function. Although the physiology and potential morbidity associated with diastolic dysfunction is well recognized as a component of pediatric heart disease, consistently accurate characterization in individual patients remains elusive for echocardiographers [1]. There are several reasons for this: (i) diastolic disease is felt to be relatively rare in children; (ii) there is no single measure that adequately describes diastolic function (in contrast to ejection fraction, which is a simple method to characterize systolic function); (iii) echocardiographic measures of diastolic function vary significantly with age and heart rate in children in the absence of true diastolic disease; and (iv) patterns of variation in these measures that effectively characterize diastolic disease in adults are less reliably discernable in children [1]. Despite these challenges, the utility of diastolic assessment in children using echocardiography continues to evolve, and this chapter focuses on the current techniques of diastolic function assessment. Diastole is the phase of the cardiac cycle during which ventricular filling occurs (Figure 8.1). It is defined as the time period beginning at end‐ejection (closure of the semilunar valves) and extending until the atrioventricular (AV) valves are closed. Diastole can be further divided into four components: Many factors influence ventricular diastolic filling, including ventricular systolic function, AV valve function, rate of ventricular relaxation, the compliance or stiffness of the atrial and ventricular muscle, atrial systolic function, the loading or volume conditions of the chambers, intrathoracic pressure changes with respiration, and heart rate and rhythm. All of these factors influence the observed diastolic velocity profile, and so must be considered in the analysis of blood inflow patterns. In the structurally normal heart, the intrinsic properties of the atria and ventricles have the greatest impact on diastolic filling, and although they are linked, we will discuss each chamber separately to better characterize the physiology. There are two main components that influence diastolic ventricular filling: active relaxation and chamber compliance. Relaxation is considered “active” because the ventricular myocardium must uncouple from the contracted state at end‐systole to initiate diastolic filling. This requires an influx of intracellular calcium to release actin–myosin bridging, and the potential energy stored in the contracted chamber generates an intraventricular pressure gradient to create a “suction effect” within the cavity that facilitates early diastolic ventricular filling. Recent investigations have helped elucidate this complex process, particularly for the left ventricle, where myocardial shortening and expansion occur with helical rotation, so that the ventricle is twisting and untwisting during the cardiac cycle (Figure 8.2) [2]. Maturational changes in rotational mechanics evolve from fetal [3] to early infancy [4] through young adulthood [5,6] that likely help explain age‐related variations in early diastolic filling patterns in children that are unrelated to cardiac disease. Left ventricular untwisting again attenuates in healthy older adults, with a concomitant decrease in early filling as part of “normal aging” [7]. Early ventricular filling is also facilitated by restoring forces (the ventricle returning to its native shape after systolic contraction) and ventricular load (the force of blood being propelled into the ventricle with AV valve opening). Figure 8.1 Simultaneous recordings of left atrial pressure (LAP), left ventricular pressure (LVP), and mitral valve Doppler inflow tracings in open‐chested dogs. When LVP decreases to a value equal to LAP (the first crossover point shown as X1), the mitral valve opens and the onset of flow occurs on the mitral valve Doppler trace. Both pressures decrease rapidly after mitral valve opening, with a short duration of transvalvar pressure difference. The peak E velocity (E) occurs at the second crossover point X2, which corresponds closely in time with the left ventricular minimum pressure. Deceleration from the peak E velocity begins with the onset of the increase in left ventricular diastolic pressure (the rapid filling wave) and ends at the third crossover point X3. In mid‐diastole, the two pressures appear to be equal, increasing slowly together (diastasis), and there is no flow across the mitral valve. Left atrial pressure increases and exceeds LVP with atrial contraction, producing flow across the mitral valve at the A wave of the Doppler trace. ECG, electrocardiogram. Source: Courtois M, Kovacs S, Ludbrook P. Transmitral pressure‐flow velocity relation. Importance of regional pressure gradients in the left ventricle during diastole. Circulation 1988;78:661–71. © 1988, Wolters Kluwer Health, Inc. Figure 8.2 Ventricular twist/untwist illustration. The temporal sequence of left ventricular twist (LVT). (a) Relative rotation of the left ventricular base (red curved arrow) and apex (blue curved arrow) during isovolumic contraction (IC), ejection, and diastolic untwisting. (b) Left ventricular rotational (R) curves for the base (red line) and apex (blue line) from a normal healthy subject. The LVT (black line and arrow) is the peak systolic difference between basal and apical rotation (arrow). (c) Left ventricular rotational rate curves for the LV base (red line) and apex (blue line). The LVT rate (black line, arrow 1) is measured as the peak systolic difference of basal and apical rotational rates, and LV untwist rate (black line, arrow 2) is the peak early diastolic difference between apical and basal reverse rotations. AVC, aortic valve closure. Source: Omar AM, Vallabhajosyula S, Sengupta PP. Left ventricular twist and torsion: research observations and clinical applications. Circ Cardiovasc Imaging 2015;8:e003029. © 2015, Wolters Kluwer Health, Inc. Ventricular chamber compliance, as reflected in the change in cavity pressure with incremental increases in chamber volume, also impacts ventricular diastolic filling, particularly in later diastole with progressive inflow. Myocardial stiffness and composition/structure of the extracellular matrix largely determine ventricular compliance in the absence of congenital heart disease [8]. Chamber compliance is adversely influenced by increased hypertrophy of the ventricle, alterations in the extracellular matrix (apparent in both immature and aging myocardium), myocyte loss, and fibrosis [9]. In healthy adults, the normal aging process does not appear to significantly impact systolic function but does result in collagen accumulation/cross‐linking of collagen in the cardiac extracellular matrix and myocyte loss [10] with a significant reduction in chamber compliance (Figure 8.3) [9]. The atrium facilitates filling of the ventricle with three distinct phases (Figure 8.4) that describe the different physiologic/functional roles of the atrium: (i) as a “reservoir” during ventricular systole, when venous return fills the atrium against a closed AV valve (from the left atrial (LA) minimum volume at end‐diastole to the maximum volume at end‐systole); (ii) as a “conduit” during early diastole, when the atrium empties into the left ventricle along the pressure gradient that develops with ventricular relaxation/AV valve opening (from the LA maximum volume to the volume prior to atrial contraction); and (iii) as a “contractile” pump during late diastole, when the atrium actively contracts to pump blood into the ventricular cavity (from the LA volume immediately before atrial contraction to the LA minimum) [11]. Atrial reservoir function is influenced by atrial relaxation and compliance as well as ventricular systolic function, because active AV valve descent during ventricular contraction augments atrial filling. Atrial conduit function is primarily influenced by atrial compliance and ventricular relaxation/early filling properties of the ventricle, while atrial contractile function is influenced by the atrial volume status in late diastole, contractility properties of the atrium, and late diastolic ventricular compliance. Ventricular filling during atrial contraction is augmented in young infants and provides important additional preload beyond the 15–30% seen in older children and adults [12]. This is likely related to the previously mentioned immature ventricular myocardial structure and mechanics. The same factors that influence ventricular filling are important in atrial filling as well; in addition, AV valve competence and venous vessel compliance play a significant role. Figure 8.3 Diastolic pressure–volume relationships. (a) Diastolic pressure–volume curves for different age groups: G21−34 (21–34 years), G35−49 (35–49 years), G50−64 (50–64 years) and G≥65 (≥65 years). Note the steeper slope of the pressure–volume curve for G50−64 and G≥65 compared to G21−34, suggesting a stiffer ventricle. Although the left ventricular (LV) chamber compliance in G50−64 and G≥65 appeared to be identical, LV pressure–volume curves shifted leftward with increasing age. (b) Diastolic transmural pressure–volume curves for G21−34, G35−49, G50−64 and G≥65. Note the steeper slope of the transmural pressure–volume curve for G50−64 and G≥65 compared to G21−34, also suggesting a stiffer ventricle. PCWP, pulmonary capillary wedge pressure. Source: Fujimoto N, Hastings JL, Bhella PS, et al. Effect of ageing on left ventricular compliance and distensibility in healthy sedentary humans. J Physiol 2012;590:1871–80. © 2012, John Wiley & Sons. Figure 8.4 Schematic representation of the left atrial volume curve and measurement of left atrial volume. AVC, aortic valve closure; AVO, aortic valve opening; ECG, electrocardiogram; LAVmax, maximum left atrial volume; LAVmin, minimum left atrial volume; LAVpreA, preatrial contraction left atrial volume; MVC, mitral valve closure; MVO, mitral valve opening. Source: Mika M, Kanzaki H, Amaki M, et al. Impact of reduced left atrial functions on diagnosis of paroxysmal atrial fibrillation: results from analysis of time‐left atrial volume curve determined by two‐dimensional speckle tracking. J Cardiol 2011;57:89–94. © 2011, Elsevier. Systemic and pulmonary venous inflow is pulsatile and can generally be separated into systolic and diastolic phases as they fill the atrium. The systolic phase occurs during ventricular systole when atrial pressure drops as the atria relax after atrial contraction. As previously mentioned, there is active apical displacement of the AV valve annulus during ventricular systole, which creates a suction effect in the atria, with resultant augmentation of venous emptying into the atria. As atrial filling continues in later systole against a closed AV valve, atrial pressures rise and venous inflow decelerates. The diastolic phase of venous inflow is initiated with AV valve opening in early diastole, as this causes a sudden decrease in atrial pressure. Diastolic venous inflow begins to decrease with increasing ventricular pressure as the ventricular chambers fill. With atrial contraction, there is a sudden increase in atrial pressure and cessation of venous inflow. If atrial pressures are elevated at the time of atrial contraction, significant reversal of flow from the atria into the veins can be seen and has been described as the atrial reversal phase of venous inflow. The current understanding of diastolic function and characterization of diastolic disease have mostly developed through investigation of intracardiac diastolic blood flow patterns using Doppler echocardiography [13]. The majority of work done on both adults and children has involved analysis of mitral and pulmonary venous inflow Doppler patterns [14–17], although interest in right ventricular (RV) diastolic function has grown recently. Pulsed Doppler analysis of mitral inflow velocities is straightforward and easily incorporated into a routine complete echocardiographic study. The best position for Doppler analysis is the apical four‐chamber view, where the Doppler signal can be aligned nearly parallel to mitral inflow, allowing accurate quantitation of peak velocities. Sample volume position is also critical, with the peak velocities generally best recorded distal to the mitral annulus near the tips of the valve leaflets. Both peak E and peak A velocities are significantly decreased as the sample volume is moved into the LA. The use of color Doppler can help better align both the Doppler cursor and sample volume positions. Several different indices of left ventricular diastolic function can be derived from the mitral valve Doppler inflow trace (Figure 8.5) [18]. These include diastolic time intervals, peak velocities during early filling and with atrial contraction, area filling fractions, velocity and area ratios, and peak filling rate. The most commonly used diastolic time interval is the isovolumic relaxation time (IVRT), which is the time from aortic valve closure to the initiation of diastolic flow across the mitral valve. It can be measured from the aortic closure component of the second heart sound as recorded on a phonocardiogram to the onset of diastolic flow velocity from the mitral valve Doppler tracing. It is also possible to measure this interval using continuous‐wave Doppler positioned at the apex with the Doppler cursor angled to record both left ventricular outflow tract velocities in systole and left ventricular inflow velocities simultaneously from a five‐chamber view. The time from cessation of aortic forward flow to the onset of diastolic mitral inflow is the IVRT. This index is regarded as relatively heart rate independent. In patients with impaired left ventricular relaxation, IVRT is prolonged; conversely, in patients with abnormal left ventricular compliance and elevated LA pressures, it may be shortened. The deceleration time during early filling from peak E velocity to cessation of flow has also been used to describe the time course of relaxation, with more prolonged deceleration correlating with delayed relaxation. The duration of diastolic flow varies with heart rate, however, so deceleration time shortens with increasing heart rate irrespective of the diastolic properties of the heart [19] and is generally not a clinically useful measure in tachycardic infants and children. Figure 8.5 Typical mitral inflow Doppler during childhood. Note the dominant peak E and smaller peak A velocities. The velocity time integrals of the E wave (E VTI) and A wave (A VTI) are outlined. Deceleration time (DT) is measured from the peak E velocity to cessation of early diastolic flow (arrows). Recommendations for measures to assess AV valve inflow include E‐wave and A‐wave velocities, A‐wave duration, deceleration time, IVRT, and calculation of the E/A ratio [20]. Studies using pulsed‐wave Doppler to assess pulmonary venous inflow have described a variety of patterns in different disease states; information obtained using this technique appears complementary to mitral inflow Doppler analysis and has added to the understanding of left ventricular diastolic function [21–24]. To obtain pulmonary venous inflow Doppler measurements, the pulsed‐wave sample volume is placed in the right or left upper pulmonary vein from an apical four‐chamber window. From this view, flow as it enters the LA can be aligned parallel to the Doppler beam using color Doppler. The sample volume should be placed as far distally into the vein as possible, preferably 1–2 cm distal to the orifice, for the most accurate and reproducible data. The closer the sample volume is placed to the orifice of the vein, the more turbulent and less distinct the Doppler signal becomes. In the uncommon pediatric patient with a suboptimal apical four‐chamber view, the parasternal, subxiphoid, and/or suprasternal views may provide visualization and alignment with pulmonary venous inflow. Transesophageal imaging also provides an excellent window for evaluation of pulmonary venous inflow. Measurements performed on the pulmonary venous inflow Doppler trace include peak systolic velocity, peak diastolic velocity, the velocity time integral during the systolic and diastolic phases compared with the total area under the curve, and systolic/diastolic velocity and velocity time integral ratios (Figure 8.6). The systolic pulmonary venous flow is frequently biphasic in infants and young children and likely represents dissociation between atrial relaxation and mitral annular displacement. The highest systolic velocity is usually measured. Finally, the velocity and duration of atrial reversal have been measured and found to be important in differentiating diastolic Doppler patterns in the face of elevated LA and left ventricular end‐diastolic pressure (see section “Patterns of diastolic dysfunction”). Right heart filling can also be assessed by Doppler echocardiography. Indices of diastolic function used for mitral and pulmonary vein inflow can be applied to the tricuspid and systemic venous inflow Doppler patterns, and appropriate positioning of the Doppler cursor remains important. Right ventricular diastolic function can be difficult to assess by inflow Doppler analysis of tricuspid and systemic venous inflow, however, because of variable preload with normal respiration. There can be dramatic variations in RV filling with changes in intrathoracic pressure during the respiratory cycle. Inspiration lowers intrathoracic pressure and augments systemic venous emptying into the right atrium (RA), and this alters the patterns of both systemic venous emptying and tricuspid inflow that are unrelated to the diastolic properties of the RV. Forward systemic vein inflow is augmented and tricuspid inflow velocities increased with inspiration, and these flows are decreased with exhalation/positive‐pressure ventilation when intrathoracic pressure increases. The tricuspid inflow peak E velocity increases by 26% and peak A by 20% with inspiration in normal children. Figure 8.6 Pulmonary venous inflow Doppler. Phasic flow is characteristically seen with peaks in diastole (D) and systole (S) and deceleration of flow at end‐systole and end‐diastole. Atrial reversal of flow (AR) can be seen in late diastole with atrial contraction and may reflect elevated left ventricular end‐diastolic filling pressure. Inflow Doppler patterns are influenced by AV valve stenosis, AV valve insufficiency, pulmonary/systemic venous obstruction, and arrhythmias. When these are present, assessment of the diastolic properties of the ventricle using inflow Doppler becomes difficult and should be made with caution. Changes in inflow Doppler tracings may not reflect alterations in the intrinsic diastolic properties of the ventricular muscle as they are frequently influenced by myocardial loading conditions. The development of ultrasonic quantitative imaging of myocardial motion has improved understanding of myocardial diastolic function in both normal children and children with heart disease. The technique that has been the focus of the majority of investigation, called Doppler tissue imaging (DTI), allows regional assessment of myocardial wall velocities throughout the cardiac cycle. Specifically, pulsed Doppler assessment of longitudinal mitral, septal, and tricuspid annular motion has yielded important new observations in both children and adults, and has become a complementary technique to standard diastolic indices of function using echocardiography. Application of this technique in the analysis of mitral annular motion has provided additional insight in the noninvasive assessment of LV diastolic function [25–29]. Similarly, tricuspid annular motion as an assessment tool for diastolic RV function has been described [29,30]. Importantly, there is no significant variation in tricuspid annular motion with respiration, suggesting that this technique, unlike tricuspid inflow, is relatively preload independent [29]. The mitral early diastolic annular velocity also appears to be relatively preload independent, so that changes in intravascular volume have little effect on that velocity [25]. This is in striking contrast to mitral inflow velocities, which can change dramatically with changes in intravascular volume [27]. Given the variable volume loads that return to the heart in children with congenital heart disease, it appears that pulsed DTI may be a complementary, less load‐independent technique in assessing ventricular diastolic function in pediatric echocardiography. Ventricular myocardial contraction and expansion occur along the major (apex to base) and minor (anterior to posterior) axes. AV valve annular motion occurs along the long axis of the ventricle as the annulus is displaced toward the apex in systole and away from the apex in diastole. Early diastolic motion of the annulus appears to reflect recoil of the ventricle from a contracted state and has correlated well with other indices of relaxation in clinical studies [25,27], whereas late diastolic annular motion is likely affected by both ventricular diastolic and atrial systolic function. The apical echocardiographic window appears to be an ideal view to assess annular motion because the motion is parallel to the apex, allowing well‐aligned Doppler interrogation throughout the cardiac cycle. In addition, the apex and crux of the heart are relatively fixed in position with little translational (i.e., rocking) motion of the heart throughout the cardiac cycle. Figure 8.7 Apical four‐chamber image of Doppler cursor position to obtain pulsed Doppler tissue imaging (DTI) recordings from the mitral septal–annular and mitral lateral–annular junctions and the tricuspid medial annular (nonseptal) junction. Doppler assessment of annular motion requires a modified wall filter and decreased overall gains to display the low‐velocity, high‐amplitude signals of the myocardium while avoiding blood flow detection. This modification is available from most commercial ultrasound vendors. Annular pulsed DTI recordings are best obtained from an apical four‐chamber view with the patient in the left lateral decubitus position (Figure 8.7). Transducer angulation and position changes to maximize parallel alignment of the Doppler cursor with the plane of maximal annular motion are frequently required. The pulsed Doppler cursor generally provides the best annular velocity signals when the sample volume gate is 3–5 mm in children. In order to visualize clearly the low‐velocity spectral Doppler patterns, the Nyquist limits should be decreased to 15–30 cm/s while using the lowest wall filter settings. It also is helpful to lower the dynamic range to 30–35 dB and decrease overall gains to minimize noise around the signal. Recordings should be made with a simultaneous ECG display to correlate timing of annular motion with cardiac electrical events. Doppler tracings should be displayed at a sweep speed of 100–150 mm/s to enable separation of waveforms and assess temporal changes in myocardial wall motion. The mitral and tricuspid annuli have circumferential attachments to the ventricular myocardium. Mitral annular motion at the septal–annular junction is easily obtained because the transducer can be best maintained parallel to annular motion at the septum. It may not completely reflect left ventricular dynamics, however, because of the influence of RV function on septal motion. Lateral–annular motion probably provides a better assessment of left ventricular myocardial function, but it is harder to maintain a parallel transducer position when assessing the lateral–annular junction because of translational shifts in cardiac position during the cardiac cycle. Tricuspid annular motion, as a reflection of RV longitudinal function, is best assessed at the medial–annular (i.e., nonseptal) junction. All three positions can provide high‐quality spectral Doppler tracings that can be easily recorded and interpreted. Several diastolic measurements can be obtained from the pulsed DTI tracings of annular motion (Figure 8.8). Two diastolic waveforms are usually seen, much like the E and A waves on inflow Doppler, that represent the early diastolic annular velocity (called the Ea or e′ wave) and diastolic velocity with atrial contraction (called the Aa or a′ wave). These waveforms are seen below the baseline as the annulus moves away from the apex in diastole. A third distinct waveform is seen above the baseline during systole and represents systolic mitral annular motion toward the apex (called the Sa or s′ wave). Diastolic time intervals can also be measured. Isovolumic relaxation, the time from the end of systolic motion to the initiation of early diastolic motion, can frequently be identified. It may be associated with very low‐velocity signals that likely represent translational shifts in heart position with the transition from systole to diastole. Although pulsed DTI of both tricuspid and mitral annular motion has been found to be clinically useful, some caveats are important to remember when applying this technique. DTI velocities, just like blood flow velocities, are dependent on the angle of insonation; it is critical to interrogate the myocardial segment parallel to the wall motion in order to obtain the true peak velocities. Some myocardial segments have very low‐velocity motion, and sometimes it can be difficult to separate this motion from translational shifts in the cardiac mass during the cardiac cycle. Finally, it is important to remember that segmental wall motion patterns may not reflect global myocardial function. Left atrial volume appears to be a reliable marker of duration and severity of left ventricular diastolic disease. It is attractive because whereas Doppler indices describe diastolic function at one moment in time, LA volumes reflect chronic cumulative filling pressures over time. Left atrial volume has been well correlated with the severity of diastolic dysfunction as estimated by Doppler echocardiographic methods in the elderly; only 9% of the population with evidence of normal diastolic function had LA enlargement (defined as <30 mL/m2 in women and <33 mL/m2 in men) whereas 100% of those with estimated severe dysfunction had a significantly enlarged LA [31]. Left atrial enlargement, as evidenced by an indexed volume of >32 mL/m2, has also been shown to be a risk factor for a first cardiovascular event (atrial fibrillation, stroke, heart failure) in elderly adults [32]. Both RA [33] and LA enlargement have been correlated with mortality in the elderly, with those patients with the largest atria having the poorest survival. Abnormal LA size has also been correlated with heart disease in children with ventricular septal defects, patent ductus arteriosus, hypertrophic cardiomyopathy, and obesity, suggesting that this assessment is important in children as well [34–36]. Figure 8.8 A typical pulsed Doppler tissue imaging tracing from the mitral annulus showing the different measures that can be obtained. Two diastolic waveforms are usually seen below the baseline: the annular velocity during early diastole (Ea), and the annular velocity during atrial contraction (Aa). The systolic annular velocity (Sa) is the dominant waveform above the baseline, starting shortly after the QRS complex. The isovolumic relaxation time (IR, measured as the time from the end of the systolic waveform to the beginning of the early diastolic velocity) and the isovolumic contraction time (IC, measured as the time from the end of the diastolic waveform with atrial contraction to initiation of the systolic velocity) are identified with arrows. Small, low‐velocity waveforms are commonly seen during isovolumic relaxation and likely represent translational changes in annular position. Table 8.1 Age‐related changes in 3D echo‐derived left atrial volume in healthy children Source: Linden K, Goldschmidt F, Laser KT, et al. Left atrial volumes and phasic function in healthy children: reference values using real‐time three‐dimensional echocardiography. J Am Soc Echocardiogr 2019;32:1036–45. © 2019, Elsevier. * P <0.05 for sex differences. BSA, body surface area; EF, ejection fraction; EV, emptying volume; F, female; M, male; Vmax, maximum left atrial volume; Vmin, minimum left atrial volume. Normal echo values for LA volume in children have been established and appear to vary with patient size (Table 8.1) [37–40]. Healthy infants and young children have smaller LA volumes when indexed by body surface area (BSA) compared to older children and adolescents, with cut‐off values for enlargement in older children and adolescents matching adult criteria (>34 mL/m2). Unfortunately, normal values for RA volumes in children have not been established.
CHAPTER 8
Diastolic Ventricular Function Assessment
Introduction
Definition and physiology of diastole
Ventricular diastolic physiology
Atrial physiology
Echocardiographic techniques for assessing diastolic function
Doppler echocardiography
Mitral inflow
Technique
Measurements
Pulmonary venous inflow
Technique
Measurements
Tricuspid and systemic venous inflow
Caveats
Doppler tissue imaging
Technique
Measurements
Limitations
Left atrial volume
0–24 months (n = 55) (M = 24, F = 31)
>2–5 years (n = 82) (M = 46, F = 36)
>5–10 years (n = 130) (M = 60, F = 70)
>10–14 years (n = 95) (M = 53, F = 42)
>14–18 years (n = 70) (M = 44, F = 26)
P value
Vmax, mL:
M
8.7 (5.1; 12.7)*
16.2 (14.3; 19.3)
25.9 (21.6; 29.1)*
37.4 (30.2; 48.6)*
51.7 (44.5; 62.1)
<0.0001
F
5.2 (3.3; 7.3)*
15.1 (12.2; 18.0)
22.2 (18.0; 27.4)*
31.2 (26.5; 40.6)*
42.1 (36.3; 50.8)*
<0.0001
Overall
6.2 (3.4; 9.3)
15.7 (13.4; 18.8)
23.9 (19.9; 28.4)
33.8 (28.2; 45.0)
48.9 (41.2; 58.6)
<0.0001
Vmin, mL:
M
2.5 (1.9; 4.3)*
5.6 (4.6; 6.8)*
9.0 (7.7; 10.9)*
13.6 (10.3; 17.8)*
19.5 (14.5; 23.2)*
<0.0001
F
1.8 (1.2; 2.7)*
4.7 (3.7; 6.1)*
7.8 (6.3; 10.0)*
10.7 (9.2; 15.0)*
14.5 (11.9; 18.3)*
<0.0001
Overall
2.2 (1.2; 2.9)
5.4 (4.1; 6.6)
8.3 (6.7; 10.5)
12.6 (9.6; 16.6)
18.0 (13.6; 22.0)
<0.0001
Total EV, mL
M
5.7 (3.1; 7.9)*
10.2 (9.4; 12.8)*
16.5 (14.2; 19.4)*
24.1 (19.3; 29.7)*
33.6 (29.1; 37.9)*
<0.0001
F
3.3 (2.1; 4.7)*
10.5 (8.4; 12.4)*
14.4 (11.7; 17.7)*
20.0 (17.1; 25.0)*
27.4 (25.3; 34.1)*
<0.0001
Overall
4.2 (2.2; 5.8)
10.4 (9.3; 12.6)
15.1 (13.1; 18.6)
21.7 (18.0; 28.1)
31.4 (26.8; 36.3)
<0.0001
Total EF, %
M
64.1 (62.2; 69.0)
66.1 (62.7; 69.0)
64.2 (61.3; 66.6)
63.1 (61.3; 66.5)*
63.1 (60.6; 65.3)*
0.011
F
62.1 (60.0; 69.0)
67.6 (64.0; 70.4)
64.5 (62.1; 67.2)
63.9 (61.4; 66.6)*
64.7 (62.8; 66.2)*
0.0063
Overall
63.5 (60.7; 69.0)
66.8 (63.7; 69.7)
64.3 (61.7; 66.8)
63.4 (61.4; 66.6)
63.8 (61.3; 65.7)
0.0002
Vmax/BSA, mL/m2:
M
21.0 (14.4; 26.9)*
24.2 (21.2; 29.8)
25.9 (22.8; 30.7)
28.5 (22.6; 32.9)*
28.8 (24.8; 34.5)
<0.0001
F
14.4 (12.8; 17.2)*
24.3 (19.0; 27.3)
24.9 (20.7; 28.5)
24.7 (20.8; 29.3)*
26.9 (22.5; 31.8)
<0.0001
Overall
15.8 (12.8; 21.0)
24.2 (21.0; 27.8)
25.5 (21.6; 30.1)
26.6 (21.5; 30.8)
27.9 (24.3; 33.1)
<0.0001
Vmin/BSA, mL/m2:
M
6.8 (5.0; 9.8)*
8.4 (7.2; 10.3)
9.4 (8.1; 11.8)
10.2 (8.4; 11.9)*
10.8 (8.6; 13.3)
<0.0001
F
5.3 (4.6; 6.1)*
7.4 (5.7; 9.7)
8.5 (7.1; 10.6)
8.6 (7.1; 10.8)*
9.2 (7.5; 11.5)
<0.0001
Overall
5.5 (4.8; 6.9)
8.1 (6.2; 10.0)
9.0 (7.6; 10.9)
9.6 (7.9; 11.7)
10.3 (7.7; 12.8)
<0.0001
Total EV/BSA, mL/m2:
M
14.2 (9.4; 17.2)*
16.3 (14.1; 19.2)
16.8 (14.7; 19.8)
18.0 (14.5; 21.4)*
18.3 (16.5; 20.9)
0.0009
F
9.0 (8.0; 10.7)*
16.2 (12.7; 18.5)
16.1 (13.5; 18.8)
16.2 (13.7; 17.9)*
17.9 (14.8; 20.2)
<0.0001
Overall
10.4 (8.1; 14.1)
16.2 (13.8; 18.7)
16.6 (13.9; 19.2)
17.3 (14.1; 19.5)
18.1 (15.6; 20.5)
<0.0001
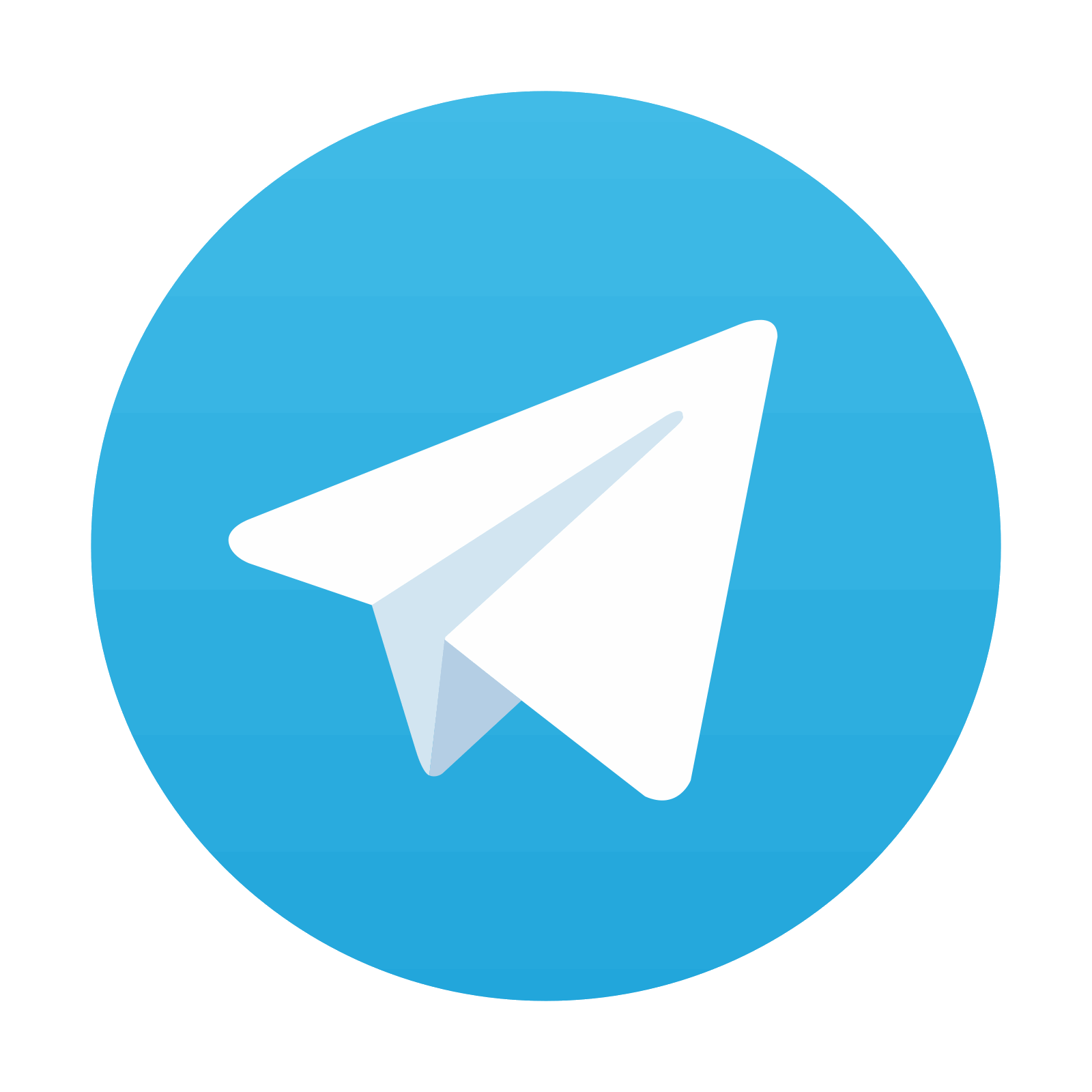
Stay updated, free articles. Join our Telegram channel
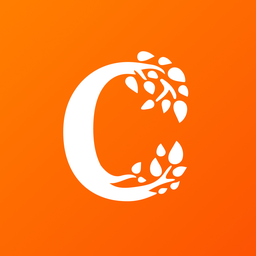
Full access? Get Clinical Tree
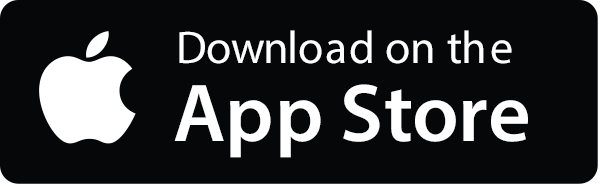
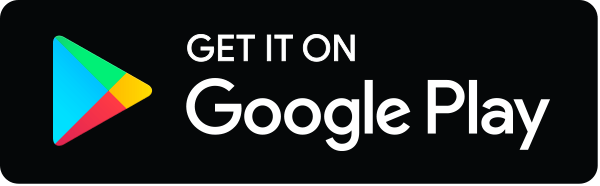