Amir H. Ashrafi1, Gabriel Altit2, and Patrick J. McNamara3 1 CHOC Children’s Hospital, Orange, CA, USA 2 McGill University, Montreal, QC, Canada 3 University of Iowa, Iowa City, IA, USA The last five decades have witnessed major advances in neonatal care with improved patient outcomes, particularly at the limits of viability. Research has primarily focused on the immature lung, thermoregulation, nutrition, and brain development, with little emphasis on heart function or vascular performance. In the neonatal intensive care unit (NICU), the usual approach to hemodynamic monitoring and decision‐making is based on a limited clinical assessment with principal emphasis placed on mean arterial pressure [1]. Recent studies highlight that treatment of low mean arterial pressure, based on a definition of gestational age equivalence, does not improve patient outcomes [2]. This has been interpreted by some commentators to mean that blood pressure (BP) is not important, however an alternative explanation is that patient assessment is inaccurate and nondiscriminatory and our approach to treatment choices is both imprecise and nonjudicious. This superficial approach to cardiovascular care places the newborn at risk of insufficient tissue oxygen delivery and end‐organ dysfunction [3]. Neonatologist‐performed echocardiography, also termed targeted neonatal echocardiography (TNE), has been utilized since the 1990s, with a significant increase over the last decade [4,5]. The primary goal of TNE is to enhance noninvasive hemodynamic assessment, guide therapeutic interventions, and ultimately improve the outcomes of newborns with hemodynamic instability. The use of echocardiography in augmenting diagnosis and tailoring therapy has become the standard of care in NICUs. From a conceptual perspective, there are some similarities to the use of point‐of‐care ultrasound in many emergency departments and other clinical care locations. The success and rapid growth of TNE in the NICU has stemmed from close collaboration between the pediatric cardiology and neonatal hemodynamic communities. International guidelines, including those from the American Society of Echocardiography (ASE), the European Association of Echocardiography (EAE), the Association for European Paediatric Cardiologists (AEPC) [1], the British Congenital Cardiac Association (BCCA) [6], and the European Society for Pediatric Research (ESPR)/European Society for Neonatology (ESN) [7], recommend the first echocardiogram in a newborn include a comprehensive anatomic assessment. Any child with suspected structural heart disease requires initiation of appropriate therapies and formal evaluation by a pediatric cardiology team. Accurate interpretation of echocardiography and formulation of a diagnostic impression requires a comprehensive understanding of cardiovascular biology, physiology, and pharmacologic treatments. Furthermore, training must be extensive and in alignment with the current guidelines [1,8]. Assessment of hemodynamics in the immediate postnatal period requires consideration of the physiologic transition into an extrauterine environment. These factors must be considered within the context of conditions unique to the neonatal period, including pathologic states impacting the maternal–fetal unit and nuances related to the spectrum of gestational maturation. The field of neonatal hemodynamics is an actively evolving subspecialty within the field of neonatology. The role of hemodynamic expertise is to augment the longitudinal physiologic information gathered at the bedside to enhance diagnostic precision and facilitate an enriched approach to the management of cardiovascular conditions impacting newborns with structurally normal hearts. The neonatal hemodynamics consultant must work closely with the pediatric cardiology team and echocardiography laboratory to provide joint oversight of the quality improvement and educational programs and liaise closely on matters related to clinical operation. The neonatal hemodynamic consultation process may be summarized into three major phases. First, the practitioner should perform a thorough clinical review including, but not limited to, the clinical history, physical examination, laboratory investigations, mechanical ventilation strategies, and medications that impact cardiovascular health. Second, and the primary focus of this chapter, the physiologic information obtained from a bedside echocardiogram (TNE) is carefully interpreted within the context of the clinical evaluation. Essential principles of a comprehensive TNE evaluation include: (i) recognition of deviations from the normal cardiac anatomy, which prompts a comprehensive echocardiogram performed and interpreted by the echocardiography laboratory; (ii) acquisition of relevant images in a systematic manner; (iii) completion of measurements according to the standards described by international guidelines on echocardiography [1,9]; (iv) completion of a report that includes information regarding anatomic surveillance and relevant hemodynamic measurements; and (v) archiving relevant images in a format that aligns with the diagnostic report and that is suitable for future audit and quality assurance. Finally, the information gathered during the clinical assessment and echocardiography scan is integrated and medical recommendations are provided, including: (i) completing a diagnostic impression; and (ii) providing a therapeutic plan with longitudinal follow‐up when warranted. Clinical recommendations and subsequent follow‐up should be based on the integration of clinical, biological, and echocardiographic information. During fetal life, gas exchange occurs at the level of the placenta, with oxygenated blood reaching the right atrium (RA) via the umbilical vein through the ductus venosus. The left atrium (LA) is underfilled secondary to the relatively low, but essential, pulmonary vascular flow in the context of constricted pulmonary vasculature. Oxygenated blood flow from the RA reaches the LA via shunting through the foramen ovale. A fraction of RA preload reaches the right ventricle (RV) and is ejected into the pulmonary artery (PA). In turn, most of this blood flow is redirected to the systemic circulation via the ductus arteriosus, secondary to the high pulmonary vascular resistance (PVR) and the relatively lower systemic vascular resistance (SVR). Indeed, the fetal aorta is connected by two fetal umbilical arteries to the low‐resistance vascular system of the placenta. Hence, the foramen ovale and ductus arteriosus shunts are described as “right‐to‐left” or “pulmonary‐to‐systemic” during fetal life. Mechanisms ensuring ductal patency include secretion of prostaglandins by the placenta and the ductus itself, as well as the relatively low fetal arterial oxygen content. Following birth, loss of the placenta leads to a drastic increase in SVR and drop in circulating prostaglandins, and the exposure of the alveoli to atmospheric oxygen leads to an acute drop in PVR. Lung expansion results in mechanical stretch of the intrapulmonary vasculature, which contributes to the fall in PVR. The subsequent exposure to increased oxygen tension triggers a cascade of endothelial mechanisms leading to the secretion of endogenous nitric oxide [10,11]. These mechanisms, compounded by the loss of the placental prostaglandins and rise in oxygen content, result in progressive constriction of the ductus arteriosus (DA) in more mature newborns [12] and alterations of blood flow through the fetal channels. Indeed, increased LA filling secondary to rising pulmonary venous return results in the displacement of the anatomic flap of the foramen ovale, functionally closing the interatrial communication. The pattern of flow through the DA progressively reverses as PVR drops. The shunts through the fetal channels become progressively left‐to‐right before their expected closure. In patients with persistent ductus arteriosus (PDA), sustained left‐to‐right transductal flow may negatively impact end‐organ perfusion, particularly cerebral perfusion for the preterm neonate [13]. The neonatal myocardium is uniquely different from that of the pediatric or adult patient in several ways. Structurally it consists of approximately 30% contractile tissue compared with approximately 60–70% in the mature heart. Neonatal cardiac myocytes are round, relatively short, and disorganized (Figure 45.1). The myofibrils are less dense and more likely to be distributed along the periphery of the cell, resulting in the centralization of disorganized mitochondria and nuclei [14]. These features partially explain the unique afterload sensitivity of the newborn’s heart [15,16]. The greater susceptibility of the RV versus the LV to afterload is particularly relevant during the transitional period. The mechanisms governing relaxation of the immature myocardium are poorly understood but also substantially different from the mature heart. The newborn myocytes lack transverse tubules, are smaller, and have a greater surface area‐to‐volume ratio [17]. Consequently, they are more reliant on trans‐sarcolemmal fluxes of calcium for contraction and relaxation [18]. In addition, studies suggest that neonates have higher total collagen content and a higher ratio of type I to type III collagen, resulting in a rigid and less compliant myocardium. In combination, these features partially explain the uniquely altered neonatal Frank–Starling curve and limited response to preload [19]. In addition, the immature myocardium, particularly the RV, poorly tolerates increased afterload. This limited capacity to augment cardiac output with ventricular filling means that heart rate and the force–frequency relationship are critical mechanisms for increasing cardiac output. Figure 45.1 Diagram showing decreased number and size of contractile units, transverse tubules, and L‐type Ca2+ channels in the myocardium of the neonate compared with pediatric patients. The transition to extrauterine life is accompanied by a significant increase in metabolic responsibility requiring the LV to increase its output by approximately 300% [20]. As a result, the LV is operating at near maximum capacity with limited ability to further enhance its output if needed [21,22]. The acute rise in postnatal SVR, along with the presence of a PDA, exposes both ventricles to an acute rise in afterload. When this is combined with an acute decrease in placental preload to the RV, the newborn is at risk of developing myocardial dysfunction, a low cardiac output state (LCOS), and hypotension. Failure of a normal postnatal transition is particularly common in premature infants. Prospective echocardiograms identified LCOS in 35% of infants born before 30 weeks and 61% of those born before 27 weeks [23]. Echocardiography assessment of cardiac output, heart function, and shunt physiology is a critical adjunct to the clinical assessment of the transitional process. Abnormal postnatal hemodynamics are commonly seen when the transitional circulation is complicated by structural heart disease, prematurity, perinatal asphyxia, sepsis, hemorrhage, or respiratory failure [24]. The disturbed perinatal transition may directly impact myocardial function [25] due to an imbalance between oxygen delivery and oxygen demand, persistent acidosis, abnormal calcium homeostasis [26], and/or hypoglycemia. The LV may be further impacted by high afterload, which may occur in the context of a catecholamine stress surge or exogenous inotropic support, as well as the fluid overload that is often present due to concomitant acute kidney injury, syndrome of inappropriate antidiuretic hormone secretion, and/or aggressive fluid resuscitation. All these hemodynamic disturbances may be exacerbated by abnormal hormonal responses, such as adrenal insufficiency from adrenal gland injury [27] or abnormal thyroid hormonal secretion from pituitary hypoperfusion or as a potential side effect of dopamine [28,29]. Finally, hemodynamic homeostasis may also be altered by sedatives and anti‐seizure medications. These complex cardiovascular intricacies must be interpreted acknowledging the high rate of altered or absent cerebral autoregulation in newborns and particularly in premature newborns [30]. The acquisition of images should be systematic and measurement techniques standardized to enhance diagnostic precision. Comprehensive TNE evaluation should include assessment of ventricular systolic and diastolic performance, right and left heart volume/pressure loading, RV and LV outputs, pulmonary arterial hypertension and pulmonary vascular resistance, and shunt physiology. Image optimization is essential to minimize measurement or analytic error. The following sections provide an overview of measurements that may be included in a comprehensive TNE assessment. The approach to echocardiographic evaluation of RV function is detailed in Chapter 9. The following is a brief summary of the pertinent measurements used in the course of a TNE. Due to its complex architecture, calculation of RV ejection fraction by 2D echocardiography is unreliable; however, the RV FAC captures the 2D fraction in surface area of the RV at end‐systole compared to end‐diastole (Figure 45.2a and b). Importantly, normative FAC data change according to gestational age and postnatal chronological age, and can be as low as 26% in the most preterm infants [31]. Normative data for RV functional assessment have been published in the term newborn [32]. Some authors have also described the use of a modified inflow–outflow RV view (Figure 45.2c), also known as the apical RV three‐chamber view, to measure FAC [32]. Inter‐reader variability regarding FAC in apical four‐chamber and three‐chamber views has been reported to be only moderate [31,32]. Neonatal patients frequently have suboptimal views, making the tracing of the endocardial border challenging. Tricuspid annular plane excursion is a commonly used measure to quantify systolic contraction of the RV in the longitudinal direction [33]. It is quantified from the lateral tricuspid valve annulus by M‐mode with the line of interrogation crossing through the apex of the RV (Figure 45.3) [34]. TAPSE has been associated with prognosis in neonates with pulmonary hypertension [35–38] and hypoxic‐ischemic encephalopathy [39]. Normative values for TAPSE have been published for term [32,40,41] and preterm newborns [41]. A major advantage of TAPSE is its high (>90%) inter‐ and intraobserver agreement in the neonatal population [42]. Figure 45.2 Apical four‐chamber view with endocardial borders of the right ventricle (RV) (a) traced in blue at end of systole and (b) traced in yellow at end of diastole for evaluation of the RV fractional area of change (FAC). (c) Apical three‐chamber inflow–outflow RV view. RV FAC is calculated by the following equation: RV FAC = 100 × (RVEDA ‐ RVESA)/RVEDA. Right ventricular output is ejected in a peristaltic motion towards the RV outflow tract (RVOT) [33,43]. A surrogate measure of RV performance is the pulsed‐wave (PW) Doppler interrogation of the RVOT quantifying its blood flow velocity. The area under the velocity–time curve, or the velocity–time integral (VTI), represents the distance that a column of blood travels during one cardiac cycle, also known as the stroke distance. When the cross‐sectional area (CSA) of the blood column is multiplied by the VTI, it produces the stroke volume (SV = VTI × CSA) [44]. The RVOT is measured in the parasternal view at the attachment of the pulmonary valve at end‐systole (Figure 45.4) [1]. Therefore, echocardiography is used to estimate RV cardiac output by the following equation: Normative RVO values for term and preterm newborns have been published [45]; however, echocardiography assessment of cardiac output is challenged by numerous limitations [46]. The formula to calculate the CSA assumes a circular pulmonary valve annulus, which is anatomically incorrect. Further, inaccuracy in this measurement will be squared and, therefore, compound the error. Furthermore, Doppler measurements occur in the center of the vessel where blood travels at its highest velocity, leading to an overestimation as the blood around the periphery travels more slowly [47]. The latter is relevant in patients with RVOT dilation, or turbulence of flow. It is important to ensure that PW Doppler, and not continuous‐wave (CW) Doppler, is obtained at the same site where the diameter measurements are obtained. The angle between blood flow direction and line of insonation should be minimized to avoid underestimation of VTI. Furthermore, there are natural variations in stroke volume during the respiratory cycle, with the highest superior vena cava flow during inspiration [48–50]. To minimize this influence, it is advised to average VTI calculations over three to five cardiac cycles. Also, inherent to neonates, length measurements can be inaccurate, hence it is reasonable to index cardiac output to body weight [7]. Despite the lack of data regarding the accuracy and reproducibility in the neonate, RV output estimates may be a useful measurement for longitudinal evaluation of RV performance. However, more studies are needed. Figure 45.3 M‐mode through the lateral tricuspid valve annulus from the apical four‐chamber view showing downward longitudinal displacement of the tricuspid valve. Figure 45.4 Echocardiographic evaluation of right ventricular output requires (a) pulsed‐wave Doppler at the tip of the pulmonary valve for assessment of stroke distance by velocity–time integral and (b) the right ventricular outflow tract diameter from a parasternal view at the peak of systole during opening of the pulmonary valve. The approach to echocardiographic evaluation of pulmonary arterial pressure is detailed in Chapter 46. The following is a brief summary of the pertinent measurements used in the course of a TNE. Pulmonary artery pressures may be estimated by echocardiography using the tricuspid regurgitation (TR) jet velocity plus an assumed right atrial pressure (5 mmHg) [51,52]. However, this method should be used only when a full Doppler spectral “envelope” is available. The TR jet velocity may be translated to pressure gradient by the modified Bernoulli equation (pressure gradient = 4 × velocity2) [53]. One of the major limitations of this measurement is that it is commonly absent or may be underestimated in patients with severe RV dysfunction, which may lead to diagnostic error if not considered. In the presence of mild pulmonary valve regurgitation, a similar approach can be used to estimate the mean PA pressure by measuring the end‐diastolic peak Doppler gradient between the main pulmonary artery and the RV [54,55]. The tricuspid valve systolic–diastolic duration (TVSD) ratio has also been described as a marker of systolic function in healthy neonates and is measurable even in the absence of a complete TR jet [43]. An elevated TVSD ratio is associated with poor clinical outcomes in the pediatric pulmonary hypertension population [56]. The direction of flow across the PDA may provide a simple and reliable surrogate estimate of PA pressure. For example, exclusive right‐to‐left shunt across the PDA suggests suprasystemic PA pressure; bidirectional flow across the PDA suggests approximately systemic PA pressure; left‐to‐right flow suggests subsystemic PA pressure. The interpretation of transductal shunt directionality must consider underlying pathophysiology. For example, patients with profound systemic vasodilation (e.g., septic shock) may have unrestrictive right‐to‐left transductal flow due to low SVR, which can be misinterpreted as suprasystemic pulmonary hypertension. In patients with arteriovenous malformations the presence of unrestrictive right‐to‐left flow may relate to the pressure loading effect of high pulmonary blood flow rather than high pulmonary vascular resistance. Nonjudicious use of inhaled nitric oxide in these clinical situations is not likely to benefit, and may harm, these unique physiologies. In the presence of a PDA or a ventricular septal defect (VSD) and with an appropriate Doppler‐derived envelope across the shunt, PA pressures can be estimated. The PDA flow velocity‐derived gradient during systole provides information about the relationship between the systemic arterial pressure and the pulmonary arterial pressure. The VSD flow velocity‐derived gradient during systole provides information about the relationship between the systolic systemic arterial pressure and the RV systolic pressure, which acts as a reliable surrogate for pulmonary arterial systolic peak pressure. This of course, is only true in the absence of pulmonary or aortic valve disease [54,55]. The position of the interventricular septum from the parasternal short‐axis (PSAX) view may be informative regarding the presence of RV hypertension and therefore pulmonary hypertension in the absence of pulmonary valve disease. If the ventricular septum is flattened at end‐systole, this indicates that the RV pressure, and therefore the PA pressure, is increased. If the ventricular septum bows into the LV at end‐systole, this indicates that the RV pressure, and therefore the PA pressure, is suprasystemic (again in the absence of congenital heart anomalies). Subjective interpretation of interventricular septal position is not without limitations and is prone to operator‐dependent error [57]. In addition, caution is advised in patients with systemic hypertension where the LV may remain circular in the presence of elevated PA pressure. Measurement of systemic blood pressure at the time of TNE assessment is essential to ensure correct interpretation of septal wall motion. Left ventricular end‐systolic eccentricity index (EI) is a more objective measurement used to quantify septal flattening [37,38,53,58]. LV EI is the ratio of largest LV dimension (PSAX at the level of the mid‐papillary muscle) to the dimension perpendicular to the septum at end‐systole [34]. Abnormal LV EI, which is suggestive of increased RV afterload, has been described when greater than 1.23 [59]. In the context of neonatal pulmonary hypertension, RV dilation can be appreciated subjectively or objectively quantified using the LV‐to‐RV diameter ratio [54,55], which is calculated as the ratio of the distance from the septum to the LV free wall and the distance from the septum to the RV free wall at end‐systole in the PSAX view at the papillary muscle level [60]. LV/RV ratio less than 1.0 has been associated with adverse outcomes in the pediatric population [54,61]. The PAAT/RVET ratio has been described as a surrogate measure of the RV to PA coupling in neonatal populations (Figure 45.5) [54,55,62–65]. It is measured from a PW Doppler envelope of the RVOT in the parasternal views at the tip of the pulmonary valve leaflet. PAAT/RVET is influenced by both RV function and RV afterload [34,66,67]. A ratio ≤0.30 has been described as a marker of neonatal pulmonary vascular disease [34,59]. Some investigators may also use the inverse form of this ratio (RVET/PAAT (normal <3.3)), which increases with PVR or falls after pulmonary vasodilator therapy. It is important to recognize that normative values tend to change with developmental age, presumably due to the dynamic changes in RV function as well as pulmonary vascular development [62,63]. With rising RV afterload, the spectral Doppler pattern obtained at the RVOT can be seen progressively transitioning from a normal parabolic curved (“isosceles triangle”) to a “right‐angle” triangular shape as PA acceleration falls [54]. In advanced forms of pulmonary vascular disease, mid‐systolic notching may be seen which relates to the biphasic nature of pulmonary blood flow due to poor vascular compliance (Figure 45.6). PAAT is inversely correlated with right heart catheterization‐derived pulmonary arterial pressure measurements in the pediatric population [64]. Figure 45.5 Parasternal short‐axis view of the main pulmonary artery with pulsed‐wave Doppler showing a pulmonary artery acceleration time of 85 ms, a right ventricular ejection time of 224 ms, and a pulmonary artery acceleration time to right ventricular ejection time (PAAT/RVET) ratio of 0.38 (or an RVET/PAAT of 2.6). Figure 45.6 Pulsed‐wave Doppler interrogation of the right ventricular outflow tract showing: (a) a normal parabolic curve (isosceles triangle) consistent with low pulmonary vascular resistance (PVR); (b) a triangular‐shaped curve suggestive of increased pulmonary artery pressure; and (c) a biphasic‐notched flow pattern consistent with elevated PVR. The approach to cardiovascular care in most NICUs is driven by MAP with some consideration to alternate vital signs and the physical exam. Blood pressure is frequently, and incorrectly, used as a surrogate for blood flow. The most widely accepted definition of hypotension was generated by consensus opinion based on the observation that the majority of “healthy” preterm infants have an MAP greater than or equal to their gestational age (GA) in weeks [68]. It has, however, been shown repeatedly that there is a weak relationship between blood pressure and blood flow. In a group of 126 premature newborns at 5 hours of age, blood pressure and blood flow were discordant 42% of the time [69]. Another study in premature newborns less than 12 hours of age showed that the threshold of mean blood pressure less than GA incorrectly identified systemic underperfusion 29% of the time [3]. Direct assessment of the left ventricular output (LVO) may be an invaluable addition to diagnosis and treatment. For example, in neonates with low diastolic BP, TNE can help distinguish between vasodilatory shock and a PDA with significant diastolic run‐off. For patients with a narrow pulse pressure, TNE may aid in distinguishing an infant with hypertrophic cardiomyopathy from one with a pericardial effusion (Figure 45.7). The approach to echocardiographic evaluation of LV systolic function is detailed in Chapter 7. The following is a brief summary of the pertinent measurements used in the course of a TNE. In the context of normal anatomy and physiology, the LV has a bullet shape appearance and contracts preferentially in a circumferential and torsional manner, with a minimal longitudinal contribution [70]. Hence, most LV functional assessments by echocardiography account for this assumption of “bullet shape.” LV systolic function is commonly assessed by shortening fraction (SF) and/or ejection fraction (EF) as described in Chapter 7. Normal values for SF in infants and children are typically between 28% and 46% [15,71]. However, 2D‐ and 3D‐derived methods for assessment of ventricular function allow for more accurate assessment when compared with MRI‐derived functional indices [72]. Figure 45.7 Clinical algorithm for differentiating reduced preload, reduced contractility, or increased afterload in a neonate with decreased ventricular function. HOCM, hypertrophic obstructive cardiomyopathy; HTN, hypertension; LV, left ventricle; MAP, mean arterial pressure; RV, right ventricle; SVT, supraventricular tachycardia; VT, ventricular tachycardia. This is a surrogate volumetric appraisal of fiber shortening. One approach to calculate EF is to measure the LV volumes from the apical four‐ and two‐chamber views. The LV volumes are assessed by tracing the endocardial border at end‐systole and end‐diastole using the modified biplane Simpson method (Figure 45.8) [73,74]. LV EF may also be calculated using the 5/6 area × length method. This method uses the LV area at mid‐papillary level in the PSAX during systole and diastole, as well as the LV length in the apical four‐chamber view to estimate end‐diastolic volume (EDV) and end‐systolic volume (ESV) [75,76]. Normal EF in children ranges between 56% and 78% [71]. Methods for estimation of LV EF are also limited, as they assume that the LV is circular in cross‐section and conical in length (“bullet shape” appearance), which may not be correct in patients with increased systolic RV pressure. In addition, there is substantial intra‐ and interobserver variability, risk of LV foreshortening, and inaccurate endocardial tracing [73]. Neonatal patients frequently have suboptimal views making the tracing of the endocardial border challenging. In the presence of mitral valve regurgitation (MR), such as with hypoxic‐ischemic encephalopathy or a large left‐to‐right PDA with significant LV dilation, LV EF assessment by echocardiography may not reliably reflect myocardial function. Hence, the use of MR dP/dt may provide some information regarding global LV contractility. The MR jet velocity depends on the pressure gradient between the LV and LA. Given there is no significant change in LA pressure during isovolumetric contraction, the change in pressure over the change in time (dP/dt max) directly measures the force generated by the LV. Using CW Doppler over the MR, measurement of time duration between the change in velocity from 1 to 3 m/s yields the dP/dt. The normal value of MR dP/dt is 1000–1200 mmHg/s, and a value of <500 mmHg/s is indicative of severe systolic dysfunction (Figure 45.9). It is important to note that this technique is highly dependent on preload, afterload, heart rate, and myocardial hypertrophy [71,77]. Furthermore, there is no current study investigating reproducibility and accuracy in the newborn, further limiting its applicability. Figure 45.8 Assessment of left ventricular (LV) ejection fraction via the modified Simpson method by tracing the endocardial LV border in (a) end‐diastole (LVEDV) and (b) end‐systole (LVESV). The Tei index or the myocardial performance index (MPI) can be used to evaluate systolic and diastolic function simultaneously [78]. The MPI has been reported for the evaluation of LV or RV performance in neonates and children [32,78–81]. One of the unique aspects of neonatal cardiovascular physiology is that cardiac output is not always equal to systemic blood flow (SBF). Due to the persistence of fetal shunts, a left‐to‐right PDA shunt may result in LVO that is substantially higher than post‐ductal SBF [82]. Similarly, a left‐to‐right patent foramen ovale (PFO) shunt results in a RV preload, and likely RVO, being higher than systemic venous return [83]. Similarly to what has been described previously for the RV assessment, when the CSA of the blood column is multiplied by the VTI of the LV outflow tract (LVOT), it estimates the LV stroke volume (SV = VTI × CSA). LVOT diameter is usually acquired at the hinge points of the aortic valve from the parasternal long‐axis (PLAX) view at end‐systole [84,85]. Variations exist in VTI acquisition, however most experts advocate that its assessment by PW Doppler be performed in the apical five‐chamber view, at the level of the hinge point of the aortic valve (Figure 45.10) [1,7]. Image optimization is crucial to ensure the line of interrogation is parallel to the direction of blood flow and 90° to the LV outflow tract diameter. LVO is calculated using the following equation [86,87]: The limitations of output have previously been detailed. LVO estimated by echocardiography correlates strongly with MRI measurements in newborns [88]. Superior vena cava (SVC) flow has been proposed as a surrogate measure of cerebral blood flow [89]. Although controversial, studies in premature newborns have shown an association between low SVC flow and intraventricular hemorrhage (IVH) and/or death [69,90–92]. Three‐year follow‐up studies have shown that low SVC flow is associated with abnormal neurodevelopment and death [93]. SVC flow was originally described using PW Doppler recordings for VTI estimation from a subxiphoid view and with an SVC diameter estimation from a sagittal rotated high parasternal view. The SVC is consistently oval shaped and therefore prone to inaccurate CSA calculations, as described earlier. Echocardiographic SVC flow measurements have been compared with phase contrast MRI and shown to have poor correlation [94]. Therefore, caution is advised when using this measurement for clinical decision‐making. Figure 45.9 Continuous‐wave Doppler tracing from the mitral valve (MR) to obtain dP/dt max obtained between 1 and 3 m/s. If less than 500 mmHg/s this is highly indicative of left ventricular systolic dysfunction. Figure 45.10 Two‐dimensional echocardiographic assessment of cardiac output. (a) Measurement of left ventricular outflow tract (LVOT) diameter from the parasternal long‐axis view. (b) Pulsed‐wave Doppler interrogation from the apical five‐chamber view. (c) LVOT velocity–time integral tracing. Descending aorta flow (DAo), distal to the PDA, represents the SBF to the lower body [95]. Due to the challenges of reliable estimation of DAo diameter, it has been proposed that DAo VTI measurements alone be used as marker of lower body SBF [50]. The VTI of DAo flow can be assessed from a high parasternal or a low subxiphoid sagittal view using PW Doppler sampled with a minimal angle of insonation. When a PDA is present, the DAo Doppler pattern can also provide information on the magnitude and duration of left‐to‐right ductal shunting or systemic diastolic steal as seen in the context of large left‐to‐right PDA flow, arteriovenous malformation, or aortic regurgitation [96,97]. Tissue Doppler imaging (TDI) can be used to evaluate myocardial deformation using PW Doppler interrogation of a sample of heart tissue, rather than blood flow, at the base of the ventricle in proximity to the valve hinge points. Specifically, TDI assesses longitudinal motion, hence it provides a surrogate estimate average myocardial shortening and translation, from the atrial–ventricular (AV) plane towards the apex of the heart. This method assumes that the sample chosen is representative of all sections of the ventricle, which may not always be correct. Currently there are limited and conflicting data available for normative values in various gestational ages and disease states [98–101]. RV (below the tricuspid valve) and LV (below the mitral valve at the free wall or septum) TDI measures of systolic and diastolic velocities have been described [32,42,98,102–104]. More specific details about TDI are provided elsewhere. Speckle‐tracking echocardiography (STE) analysis allows for segmental systolic and diastolic functional assessment in different planes, looking at longitudinal, circumferential, radial, and rotational mechanics (see Chapter 7) [105]. Values of regional and global RV and LV strain have been reported in the normal neonatal, pediatric, and adult populations [32,58,102,106–109]. Compared to standard echocardiography techniques, STE applied on 2D‐ and 3D‐derived echocardiography images allows refinement of the assessment of RV–LV interactions and ventricular volumes, as well as global deformation assessments [108,110,111]. STE uses data obtained from the overall cardiac cycle, uncovering areas of poor or no contraction as well as dyssynchrony. The technology enables tracking of the endocardium and myocardium and derivation of 2D values of muscle deformation (Figure 45.11). This tracking also allows for 3D reconstruction of LV and RV volumes and for real‐time 3D estimation of EF and deformation of the overall heart and its segments (Figure 45.12). Strain rate correlates with peak LV elastance, a load‐independent parameter of systolic function [112]. In the context of myocardial shortening during systole (longitudinal and circumferential), the strain and strain rate values are negative, while during lengthening (diastolic relaxation) or thickening (radial contraction), they are positive [113,114]. The approach to echocardiographic evaluation of LV diastolic function is detailed in Chapter 8. The following is a brief summary of the pertinent measurements used in the course of a TNE. Assessment of LV diastolic function by echocardiography in the context of perinatal transition, including lusitropy (rate of myocardial relaxation) and compliance, remains a challenge. At a basic level, atrial shunting can provide information about underlying ventricular compliance. When shunting is bidirectional, the end‐diastolic pressures are assumed to be similar; if right‐to‐left, end‐diastolic RV pressure is assumed to be higher, and when left‐to‐right, the reverse. While this is simple, it lacks objective quantification. More sophisticated techniques described in the pediatric and adult population are described in detail in Chapter 8
CHAPTER 45
Echocardiographic Assessment of the Transitional Circulation
Introduction
Overview of targeted neonatal echocardiography
Principles and hemodynamic appraisal
Transitional physiology
Role of fetal shunts
Neonatal myocardium
Ventricular function during transition
Abnormal postnatal transition
Neonatal hemodynamic assessment by echocardiography
Assessment of RV performance and pulmonary blood flow
Right ventricular fractional area of change (FAC)
Tricuspid annular plane excursion (TAPSE)
Right ventricular output (RVO)
Pulmonary arterial pressure and vascular resistance
Regurgitation valve velocity
Shunt direction/velocity
Interventricular septal position
Pulmonary artery acceleration time to RV ejection time (PAAT/RVET) ratio
Assessment of LV performance and systemic blood flow
Left ventricular systolic performance
Left ventricular shortening fraction
Left ventricular ejection fraction
Mitral valve regurgitation dP/dt
Myocardial performance index
Left ventricular output
Superior vena cava flow
Descending aorta flow
Tissue Doppler imaging
Strain and strain rate by speckle tracking in the newborn
Assessment of LV diastolic function
Stay updated, free articles. Join our Telegram channel
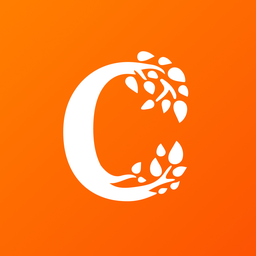
Full access? Get Clinical Tree
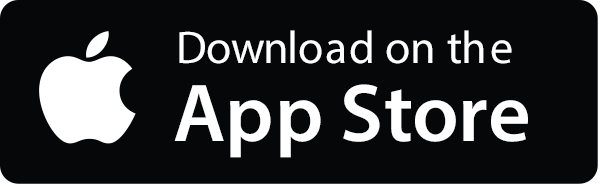
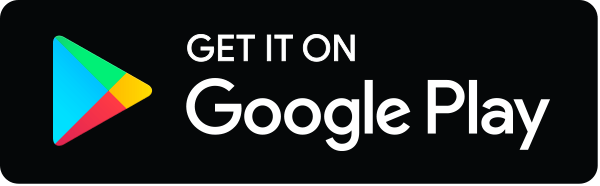