28 Duncan C. Ferguson Hypothyroidism is the most common endocrinopathy of the dog as a result of the breed-associated incidence of lymphocytic thyroiditis. Clear syndromes of congenital hypothyroidism are recognized in the neonatal horse, but the incidence and pathogenesis in the adult horse is less understood. Spontaneous hypothyroidism in the cat and other domestic species is most commonly associated with congenital disease or following thyroidectomy. Less commonly, hypothyroidism can be caused by iodine deficiency or by the ingestion of goitrogenic substances in the environment or food; goitrogenic substances are compounds that interfere with thyroid hormone synthesis by the thyroid gland. Clinical signs of hypothyroidism common to most species generally reflect the reduction in basal metabolic rate of the body and include lethargy, mental depression, weakness, inability to train, and/or nonpruritic hair loss (Ferguson, 1989a, 1993; Ferguson and Hoenig, 1991b; Ferguson et al., 1992; Peterson and Ferguson, 1990; Feldman and Nelson, 2004a). Hyperthyroidism is now the most common endocrine disorder in the cat, and is only occasionally seen in other domestic species. Hyperthyroidism or thyrotoxicosis is caused by excessive concentrations of the circulating thyroid hormones, thyroxine (T4) and triiodothyronine (T3), most commonly the result of hyperplastic or benign adenomatous malignant thyroid glands in cats and adenocarcinomas in dogs. Hyperthyroidism occurs most frequently in middle-aged to geriatric cats and discussion of therapeutic agents will focus on those used in this species. The most common clinical signs associated with hyperthyroidism, which can be directly related to thyroid hormone excess, are weight loss in spite of ravenous appetite, hyperactivity, polydipsia, polyuria, diarrhea, intermittent fever, vomiting, and symptoms of cardiovascular disease such as tachycardia and dyspnea. Often the cats shed excessive amounts of hair or the coat may be matted. Rarely, hyperthyroid cats present in a way similar to what has been called “apathetic hyperthyroidism”; the cats are lethargic and often anorectic, perhaps representing an end-stage form of the disease (Feldman and Nelson, 2004b; Ferguson and Hoenig, 1991a; Peterson and Ferguson, 1990). Therapy focuses upon either definitive treatments such as radioiodide and thyroidectomy, or, as discussed below, the administration of drugs that reduce the synthesis, secretion, metabolism, and/or action of thyroid hormone. Thyroid hormones are the only iodinated organic compounds in the body with the two major secretory products of the thyroid gland, thyroxine (L-T4) and 3,5,3′-triiodothyronine (L-T3) containing 65% and 59% iodine, respectively. The minimum iodine requirement of most animals is unknown, but the daily amount needed in the ration to prevent goiter in all animals is generally accepted to be about 1 µg/kg body weight. The daily recommended amount of iodine in the dog is 15 µg/kg and while it has not been carefully studied in the cat, it is believed to be about 100 µg/cat/day. Commercial cat foods have been shown to be quite variable in the amount of iodide provided, probably a result of the variable amount of seafood used to create the diet. Indeed, dietary iodide has been shown to be inversely related to free T4 concentrations (Tarttellin and Ford, 1994). Also, a review has questioned the adequacy of iodide in commercial cat diets before 2006, suggesting that the increased incidence of feline hyperthyroidism might have been associated with iodide deficiency (Edinboro et al., 2010). Although true nutrient requirements for this micronutrient are not well established, most commercial dog and cat food preparations include at least three to five times the minimum stated requirement for iodine when fed in recommended amounts. As a result, today, documented iodine deficiency has become a rare condition in most domestic animals. During pregnancy, the recommended minimum daily requirement for iodine is increases fourfold. Areas of iodine deficiency in North America include the Great Lakes region and eastern British Columbia (Kaptein et al., 1994; Peterson and Ferguson, 1990; Zimmerman, 2013). Ingested iodine is converted to iodide in the gastrointestinal tract and absorbed into the circulation. The dog has plasma iodide concentrations of 5 to 10 µg/dl, which are 10 to 20 times the levels in human plasma. In the thyroid gland, iodide is concentrated or “trapped” by the sodium–iodide symporter (NIS), which utilizes the sodium gradient developed by the Na+,K+-ATPase to move iodide through the basolateral plasma membrane of the thyroid follicular cell. This results in intracellular iodide concentrations that are 10 to 200 times that of serum. This process is stimulated by the interaction of thyrotropin (TSH) with G-protein-coupled TSH receptors on the surface of the follicular cell leading to the stimulation of cAMP (Figure 28.1). The transcription of NIS is stimulated by TSH or cyclic AMP. NIS is also up-regulated directly by thyroidal mechanisms reacting to iodide insufficiency or blockade of iodide transport by compounds such as perchlorate. Other tissues, including the salivary glands, gastric mucosal cells, renal proximal tubule cells, placenta, ciliary body, choroid plexus, and mammary glands, can take up considerable amounts of radioiodide in a TSH-independent fashion. Figure 28.1 Thyrotropin (thyroid-stimulating hormone, TSH)-stimulated iodide uptake and organification by the thyrocyte. Step 1: Inorganic iodide (I−) is actively translocated by the sodium–iodide symporter (NIS), using the Na+ gradient established by the Na+K+-ATPase. NIS is stimulated directly by the G protein-coupled TSH receptor, and is influenced by availability of iodide, being stimulated by iodide insufficiency and suppressed by excess iodide. After diffusing to the apical or brush border membrane, the pendrin protein carries it into the lumen of the colloid. Steps 2 and 3 Oxidation and organification: Iodide is oxidized by the thyroid peroxidase enzyme (TPO) (Step 2) and added to a tyrosine residue of preformed thyroglobulin (Tg) (Step 3) to form monoiodotyrosine (MIT) and diiodotyrosin (DIT). Step 4 Coupling: The MIT and DIT residues on Tg couple to form T3, and two DIT residues couple to form T4. Steps 2–4 are sensitive to thionamide antithyroid drugs like PTU or MMI. Step 5 Colloid resorption: Under the influence of TSH, follicular colloid–containing Tg is resorbed by the thyrocyte by pinocytosis. Step 6 Tg proteolysis: Thyroid hormones MIT and DIT are released from Tg under the influence of TSH. Step 7 Deiodination: Also stimulated by TSH at the time of secretion, deiodinase enzymes (D1,D2) are stimulated, resulting in the removal of iodide and increasing the T3/T4 ratio in the secreted pool. Step 8 Secretion: T4, T3, and rT3 are released into the bloodstream. Source: Peterson and Ferguson, 1990. Reproduced with permission of Elsevier. Diagnostically, radioactive iodide or pertechnetate (TcO4−), which, unlike iodine, cannot be organified, can be used to assess the anion transport function (uptake) by the thyroid gland. Iodide trapping can be inhibited by other anions such as thiocyanate (SCN−), NO3−, and ClO4−. Thiocyanate is a metabolic product of some naturally occurring compounds in plants and may result in goitrogenic (antithyroid) activity of the plant. Domestic animals may also be exposed to thiocyanate produced as a by-product of cigarette smoke in a household environment. Oral administration of perchlorate following the administration of a tracer dose of radioiodine can be used to diagnose congenital defects in the thyroidal organification of iodide (perchlorate discharge test) (Taurog, 1991; Kopp, 2013). Thyroglobulin (Tg), an iodinated glycoprotein with a molecular weight of 660,000 daltons, serves as a synthesis and storage site for thyroid hormone and its precursors in the thyroid follicle. After synthesis within the endoplasmic reticulum of the thyroid follicular cell, membrane vesicles containing noniodinated Tg fuse with the apical membrane and are released (by exocytosis) into the follicullar cell lumen where thyroglobulin is stored as colloid. Once inside the thyroid cell, iodide is transported to the follicular lumen by the protein pendrin, then oxidized by the enzyme thyroid peroxidase (TPO) to iodine (Figure 28.1). Pendrin is an apical transmembrane protein that transports sulfate, chloride, iodide, and bicarbonate (Larsen et al., 2003). Iodide is then incorporated by the heme protein enzyme thyroid peroxidase (TPO), which, in the presence of hydrogen peroxide, adds iodide onto tyrosine residues of Tg in a process called organification, forming monoiodotyrosine (MIT) and diiodotyrosine (DIT). TPO also forms thyroxine (T4) by coupling two DIT molecules, and 3,5,3′-triiodothyronine (T3) by coupling one MIT molecule with one DIT molecule (Burrow et al., 1989; Peterson and Ferguson, 1990; Taurog, 1991; Kopp, 2013). The reaction catalyzed by TPO is inhibited by the thionamide drugs propylthiouracil (PTU) and methimazole (MMI) and by high concentrations of iodide (the Wolff–Chaikoff effect). The intermediate product of peroxidation of iodide is either hypoiodous acid (HIO2−) or iodinium (I+). The H2O2 required is generated by NADPH oxidase. In normal humans and rats, more than 90% of thyroidal radioiodine is organified to iodotyrosines and iodothyronines within minutes of entry into the thyroid. When iodine intake is adequate, production of T4 is favored. However, in iodine-deficient states and impending thyroid failure, the intrathyroidal synthesis of T3 is preferred over that of T4. By this autoregulation, the thyroid gland produces the most active thyroid hormone (T3 is three to ten times more potent than T4) while using less iodide. Conversely, chronic iodine excess may lead to excessive storage of thyroidal hormone. The Wolff–Chaikoff effect, another intrathyroidal regulatory mechanism, is key to understanding the potential acute antithyroid effect of large amounts of ingested iodide. It is believed that, through this mechanism, the organism is protected from massive thyroid hormone release following a large dietary iodine load (Taurog, 1991; Wolff, 1989). Although the acute Wolff–Chaikoff effect is still not well understood, iodine itself has been shown to inhibit Ca++ (and NADPH-dependent H2O2) generating activity associated with NADPH oxidase, a mechanism characterized in dog, pig, and human thyroid glands. It has been proposed to be associated with autoregulation by organic iodinated compounds in the thyroid. Furthermore, this enzyme seems to be inhibited by iodinated compounds in cultured dog thyroid cells (Dugrillon, 1996; Panneels et al., 1994). In humans, the Wolff–Chaikoff effect is transient and “escape” is seen within several weeks and has been proposed to be associated with reduced iodide transport into the gland (Braverman and Ingbar, 1963). Thyroid hormone secretion is initiated as the epithelial follicular cells take up thyroglobulin in colloid droplets by a process called pinocytosis. Simultaneously, lysosomes (containing proteases and hydrolytic enzymes) migrate from the basal region of the cell and fuse with the colloid droplets (Figure 28.1). Degradation of thyroglobulin by the lysosomal proteolytic enzymes produces both the iodotyrosines (MIT and DIT) and iodothyronines (T4 and T3). Little of the released MIT and DIT enters the circulation because the iodine is removed from these compounds by deiodinase enzymes (Figure 28.1). Some of this iodine is recycled internally for iodination of new tyrosine residues in thyroglobulin, but in carnivores much iodine is released to the circulation (Belshaw et al., 1974; Kaptein et al., 1994). This inefficient thyroidal reutilization of iodine may help explain the high daily iodine requirements of the dog and cat compared to humans. Proteolysis of Tg, as described in Section Thyroid Hormone Synthesis, liberates relatively large amounts of T4, but only small quantities of T3, into the cytosol. 5’-deiodinase enzymes (D1 and/or D2) within the thyroid gland, however, can deiodinate T4 to either T3 or 3′,5′,3-T3 (reverse T3) and less-iodinated products. As a result, although the T4 : T3 ratio stored in the gland is 12 in the canine thyroid, the ratio of secreted products is 4. Production rates of the thyroid hormones in the dog have been estimated to be 8 µg/kg/day for T4 and 0.8 to 1.5 µg/kg/day for T3. In cats, T4 production rates have been estimated to be 5.6 µg/kg/day, and 0.4 µg/kg/day for T3. These production rates are greater than twice those for T4 and greater than three times the rate for T3 in humans (Kaptein et al., 1993, 1994). Thyrotropin (thyroid-stimulating hormone; TSH), a glycoprotein produced in the thyrotropes of the pituitary pars distalis, has a stimulatory effect on thyroid hormone synthesis and secretion. In addition, TSH stimulates thyroid growth, probably in conjunction with actions of the insulin-like growth factors (IGF I and II). Thyrotropin has a molecular weight of about 30,000, consisting of an α subunit (identical to the α subunit of the other glycoprotein pituitary hormones luteinizing hormone, LH, and follicle-stimulating hormone, FSH), and a β subunit, which is specific to the TSH molecule (see Chapter 26). TSH binds to a specific TSH receptor on the thyroid follicular cell membrane and stimulates adenylate cyclase, the production of cyclic AMP, and the active uptake of inorganic iodide (Figure 28.1). The TSH receptors in dog, cat, and humans have been cloned and expressed. TSH also stimulates the synthesis of thyroglobulin, its release into the colloid, and its iodination by TPO (i.e., organification). As a final step in the delivery of hormone into plasma, TSH stimulates thyroglobulin resorption and proteolysis to release T3 and T4. The thyroidal deiodinases are also stimulated by TSH (Magner, 1990; Rapaport and Nagayama, 1992; Shupnick et al., 1989). A detailed study of the hypothalamic–pituitary–thyroid–extrathyroid axis is only possible with the availability of a valid TSH immunoassays for each species. Availability of this assay for the dog has helped to demonstrate that most of the replacement dosages of L-thyroxine recommended are well in excess of those needed for suppression of endogenous TSH into the normal range. The currently available assays do not reliably distinguish between normal and low TSH concentrations making it more difficult to confirm an overdosage (Braverman and Utiger, 1991; Ferguson, 1984; Greenspan, 1994; Williams et al., 1996; Bruner et al., 1998). The canine TSH assay has also been used to evaluate thyroid status in the cat despite incomplete (35%) cross-reactivity for the assay (Ferguson et al., 2007). Measurement of an undetectable serum TSH concentration is a highly sensitive, but only moderately specific, test in support of the diagnosis the confirmation of hyperthyroidism, but should only be used in conjunction with measurement of total and/or free T4 concentrations (Wakeling et al., 2011). Following surgery, radioiodine or antithyroid medication, the measurement of a normal TSH concentration with total and/or free T4 is now recommended to evaluate treatment. There is evidence that transient hypothyroidism, associated with low total and/or free T4 and elevated TSH, is associated with usually mild and occasionally severe renal insufficiency in cats treated for hyperthyroidism (Riensche et al., 2008; van Hoek and Daminet, 2009; Daminet et al., 2014). The tripeptide TRH is produced in the paraventricular nucleus of the hypothalamus and transported to the pituitary pars distalis by the hypophyseal portal system in the pituitary stalk. In the pituitary gland, TRH binds to specific receptors on the thyrotrope cell and stimulates TSH secretion (Figure 28.2 A). In the dog, as in other species, TRH also stimulates the secretion of prolactin. The hypothalamic hormone somatostatin acts to inhibit TSH secretion and may function as a thyrotropin inhibitory factor (Reichlin, 1986). Figure 28.2 (A) Hypothalamic–pituitary–thyroid–extrathyroid axis. Please see text for details. TSH, thyrotropin; CNS, central nervous system; DA, dopamine; TRH, thyrotropin releasing hormone; SRIH, somatostatin; TSH, thyrotropin; FT3, free T3; FT4, free T4; PBP, plasma binding proteins; +, stimulation; −, inhibition. (B) Postranslational regulation of the D2 enzyme by T4 in the thyrotroph cell. Free T4 (FT4) in the circulation is available to enter the thyrotroph, and the quantity of T4 in the pituitary cell directly and rapidly inhibits the D2 enzyme, which converts T4 to T3. Posttranslational modification of the D2 enzyme by ubiquitination shortens the half-life of the enzyme and ultimately reduces the amount of T3 produced from T4. Most of the T3 binding to the nuclear receptor in the thyrotroph is derived from T4 deiodination. Occupancy of the TR results in down-regulation TSH mRNA and TSH secretion completing the negative feedback loop between FT4 and TSH. The negative feedback effect of thyroid hormones (in the free or unbound form) is the principal mechanism regulating TSH secretion. Tonic stimulation by TRH has a permissive role in TSH secretion. The pituitary thyrotope cell completely deiodinates T4 (derived from the plasma) to T3 that subsequently inhibits TSH synthesis and secretion through alteration of nuclear receptor binding, mRNA transcription, and protein synthesis. The Type II 5′-deiodinase (D2) mediates the intrapituitary conversion of T4 to T3, but, at the same time, T4 inhibits D2 activity at a posttranslational level by shortening the enzymes cellular half-life through ubiquitination and subsequent proteasomal degradation (Figure 28.2 B). D2 and TSH are coexpressed in thyrotrophs and hypothyroidism increases D2 expression in the thyrotroph. As a result, free T4 (FT4) concentration and D2-mediated T3 production correlate negatively with expression of mRNA for TSH (Christoffolete et al., 2006). Circulating T4 taken up by the pituitary is the preferred source of T3 in the pituitary, at least in the rat (Larsen et al., 1981). In human patients with hypothyroidism, thyroid replacement therapy with L-T4 normalizes serum TSH concentrations only when the serum T4 value is high normal to slightly high; serum T3 concentration usually remains within normal range in these patients (Fish et al., 1987; Larsen et al., 1981). There is also evidence that thyroid hormones may have a direct negative-feedback effect on the hypothalamus to inhibit the release of TRH (Figure 28.2 A). Also, TSH and TRH may have “short-loop” and “ultrashort-loop” negative-feedback effects, respectively, upon the hypothalamus to inhibit TRH release. Although pulses of TSH secretion and an evening rise in serum TSH have been described in humans (possibly resulting from a fall in circadian circulating cortisol concentrations), studies in the dog and cat have failed to demonstrate such a circadian rhythm in circulating thyroid hormone concentrations (Fish et al., 1987; Larsen et al., 1981; Magner, 1990; Reichlin, 1986; Bruner et al., 1998; Chiamolera and Wondisford, 2009). Pulsatile release of TSH has been confirmed in hypothyroid but not euthyroid dogs, but there was no overlap in values. However, TSH may not be elevated in some confirmed canine hypothyroidism cases, and TSH has been shown to rise initially and eventually fall again in chronic experimentally induced hypothyroid dogs, suggesting either pituitary resistance to lack of negative feedback by thyroid hormones or reduced synthesis and secretion of TSH over time (Kooistra et al., 2000; Diaz-Espineira et al., 2008, 2009). The metabolically active thyroid hormones are the iodothyronines L-thyroxine (L-T4) and 3,5,3′- L-triiodothyronine (L-T3) (Figure 28.3). Thyroxine is the main secretory product of the normal thyroid gland. However, T3, which is about three to ten times more potent than T4, as well as smaller amounts of 3,3′,5′-L-triiodothyronine (reverse T3), a thyromimetically inactive product, and other deiodinated metabolites, are also secreted by the thyroid gland of most mammals (Figures 28.2 A and 28.3) (Belshaw et al., 1974; Ferguson, 1984; Inada et al., 1975; Kaptein et al., 1993, 1994; Laurberg, 1980). Figure 28.3 Pathways of metabolism of thyroid hormones. 5′-D, 5′-deiodinase; 5-D, 5-deiodinase. Source: Ferguson, 1984. Reproduced with permission of Elsevier. Although all T4 is secreted by the thyroid, a considerable amount (40 to 60% in the dog) of T3 is derived from extrathyroidal enzymatic 5′-deiodination of T4. Therefore, although it also has intrinsic metabolic activity, T4 has been called a “prohormone,” and its “activation” to the more potent T3 is a step regulated individually by peripheral tissues (Figures 28.3 and 28.4). The vast majority (approximately 90%) of reverse T3 (rT3) is derived from extrathyroidal sources in the dog (Belshaw et al., 1974; Kaptein et al., 1993, 1994; Larsen et al., 1981). Figure 28.4 Peripheral action of thyroid hormones. T4 and T3, in amounts proportional to their free forms (FT4 and FT3) in plasma at equilibrium with plasma binding proteins (PBP), are taken up by peripheral tissues such as liver and kidney, which have the type I 5′-deiodinase enzyme (5′-D-I). T3 from the plasma (or that derived from T4) interacts with mitochondrial receptors to rapidly increase oxygen consumption and with nuclear receptors to initiate protein synthesis. Cytosolic binding proteins (CBP) buffer the effects of intracellular hormones and provide a relatively unsaturable hormone reservoir. In the brain, pituitary, and brown fat, another isoenzyme of the 5′-deiodinase enzyme (5′-D-II) converts T4 to T3. This enzyme is regulated very differently from the type I enzyme. Source: Peterson and Ferguson, 1990. Reproduced with permission of Elsevier. The identification of three distinct types of deiodinase enzymes has underscored the importance 1981 of the regulation of T3 production in individual tissues from T4. Type I 5′-D (D1) is found in most tissues, but has its highest activity in liver, kidney, muscle, and thyroid gland. This enzyme is now known to be a selenoenzyme, requiring trace quantities of selenium for optimal activity. Muscle, although it has low enzyme activity, may produce a significant amount (approximately 60% in the rat) of the body’s T3 solely because of its large mass. The physiological role of D1 is the provision of circulating T3 during conditions of euthyroidism. This enzyme is capable of “outer-ring” and “inner-ring” deiodination (ring with amino acid moiety) and can deiodinate T4 with a high capacity and is sensitive to the thionamide antithyroid agent, propylthiouracil (PTU). Type II 5′-D (D2) is found in the CNS, pituitary, brown fat, and placenta, and its physiological role is provision of intracellular T3. Its role in contributing circulating T3 may increase with primary hypothyroidism. This enzyme acts upon T4 and other compounds with outer-ring iodine at concentrations within the physiological range and is resistant to PTU. Type III deiodinase (D3), which is a 5-deiodinase, removes only inner-ring iodines. D3 is present in placenta, CNS, skin, and fetal brain and liver and has as its physiological role inactivation of T4 and T3 through inner-ring deiodination, with preference for T3 as a substrate. Relevant to understanding the rationale for L-thyroxine therapy in hypothyroidism, hypothyroidism dramatically reduces the activity of D1 and D3 while increasing the activity of D2. Through this type of regulation, the brain may continue to obtain adequate cellular T3 levels necessary to prevent or delay neurological dysfunction resulting from T4 deficiency, while the liver reduces its production of T3, thereby leading to decreased systemic metabolism (Figure 28.4). It is interesting to note that, in rodents, D1 and D3 are increased in hyperthyroidism resulting in both increased production and degradation rates for T3. The characteristics of D1 and D2 have been examined in dogs, cats, and cattle. In cats, renal and hepatic D1 has been shown to metabolize T4 with similar Vmax and Km of the rat enzyme, but the ability of the enzyme to degrade rT3 was only about 0.2% of that of rat D1 with a Km 500-fold higher. The D1 enzyme was not identified in the cat’s thyroid gland, unlike in rat, dog, and humans. These differences would imply that feline D1 does not metabolize rT3 under physiological conditions. However, muscle D1 mRNA is stimulated by T3 treatment of lean cats, as seen in most species. D2 has been identified in human keratinocytes as well as in dog skin. Previous studies have indicated important differences in catalytic specificity between dog and human D1, in particular with respect to the 5′-deiodination of rT3. While D1 and D2 are found in the microsomal fraction of cells, D3 seems to be a plasma membrane protein, positioning it well to inactivate both excess T4 and T3 in tissues such as the placenta and brain (Figure 28.5 A) (Baqui et al., 2003; Burrow et al., 1989; Ferguson, 1988; Larsen et al., 1981; Peterson and Ferguson, 1990; Foster et al., 2000; Bianco, 2002; Hoenig et al., 2008). Figure 28.5 (A) Cellular localization of thyroid hormone transporters, deiodinases, and nuclear receptors. The D1 and D3 enzymes are plasma membrane-associated enzymes and D1 provides circulating plasma T3 to plasma and D3, or 5-deiodinase, leads to inactivation of T4 to rT3 or T3 to 3,3’-T2. The D2 enzyme is associated with the endoplasmic reticulum in tissues where it dominates (brain, pituitary, brown fat, etc.). Under euthyroid conditions, it provides intracellular T3, but can provide a larger portion of circulating T3 under hypothyroid conditions when D2 activity is increased. Important plasma membrane transporters for T4 and T3, depending upon the tissue, include monocarboxylic acid transporter 8 (MCT8) and organic acid transporter p1c1 (OATp1c1). The lipophilic hormones are also capable of diffusing through cell membranes after dissociating from thyroid binding proteins in the circulation. Once in the cytosol they also can associate with cytosolic thyroid binding protein (CTPB) until delivered to sites of action or metabolism. T3 enters the nucleus and has high affinity for the thyroid hormone receptor (TR). TR is a heterodimer with the retinoic X receptor (RXR), which binds to the thyroid-response element (TRE), leading to a change in mRNA transcription (increase or decrease) and effects secondary to altered protein synthesis. The process of deiodination continues until the thyroid hormone nucleus is stripped of its remaining iodine molecules thereby allowing iodine to recycle for hormone resynthesis (Figure 28.3). These further deiodinated metabolic products (other than T3 and T4) do not have thyromimetic activity. Evidence is accumulating that reverse T3 may have an important role in regulating thyroid T4 and T3’s activity in the central nervous system and 3,5-diiodothyronine (3-5-T2) and 3-monoiodothyronine (3-T1) may have cardiovascular effects (Ianculescu and Scanlan, 2010; Axelband et al., 2011; Dentice et al., 2013; Orozco et al., 2014). A number of nonthyroidal illnesses and drugs may affect the local tissue regulation of thyroid hormone deiodination. Other pathways of thyroid hormone metabolism include conjugation to form soluble glucuronides and sulfates for biliary or urinary excretion as well as cleavage of the ether linkage of the iodothyronine molecule (Braverman and Utiger, 1991; Burrow et al., 1989; Ferguson, 1984, 1988; Kaptein et al., 1994). With oral administration of thyroid hormone preparations, the first-pass effect must be considered, as a large quantity of hormone can be conjugated and secreted into the bile where the hormone may either be deconjugated and reabsorbed by bacteria in the large intestine, or eliminated in the feces. The intestinal pool of thyroid hormone is known to be very large. In the dog, over 50% of the T4 and about 30% of the T3 produced each day are lost into the feces. In both the dog and cat, the extrathyroidal body stores of T4 are eliminated and replaced in about 1 day, whereas stores of T3 are lost and replaced twice daily (Kaptein et al., 1993, 1994). Such fecal wastage is responsible, in part, for the higher daily replacement doses of thyroid hormone required on a per body weight basis in dogs and cats. Despite occasional reports of massive overdoses of levothyroxine products (Hansen et al., 1992), the fecal loss pathway also accounts for the low morbidity with accidental toxicoses with thyroid hormone products in dogs. Nonetheless, demonstrating interindividual differences in sensitivity, it has been reported that a dog became thyrotoxic after coprophagia of feces from another dog in the household receiving a high dose (0.8 mg) of levothyroxine twice daily (Shadwick et al., 2013). Thyroid hormones are water-insoluble lipophilic compounds. Their ability to circulate in plasma is dependent upon binding by specific binding proteins, thyroxine-binding protein (TBG) and transthyretin (TTR) or thyroxine-binding prealbumin (TBPA), as well as by albumin itself. Thyroid hormone–binding proteins provide a hormone reservoir in the plasma and “buffer” hormone delivery into tissue (Figure 28.4). TTR and possibly albumin also may serve as intermediary carriers for specific tissue uptake of the hormone by tissues (Mendel, 1989; Pardridge, 1981). The dog has a high-affinity thyroid hormone–binding protein, comparable to TBG in humans, but plasma concentrations of TBG in the dog are only 25% of those in humans. In addition to TBG, TBPA, and albumin, circulating T4 in canine plasma appears to bind to certain plasma lipoproteins. These include a high-density lipoprotein (HDL2), which migrates in the alpha1 region on the electrophoretic pattern and a very low-density lipoprotein (VLDL), which migrates in the beta region. At normal serum T4 concentrations in the dog, about 60% of T4 is bound to TBG, 17% to TBPA, 12% to albumin, and 11% to the HDL2. Thyroxine-binding globulin in the dog is not saturated until the total T4 concentration is six times the normal serum T4 values, while the other serum proteins are virtually unsaturable (Inada et al., 1975). The cat does not appear to have a high-affinity thyroid-binding protein (such as TBG) but has only TTR and albumin as serum thyroid hormone–binding proteins. Partly as a result of weaker serum protein binding, total T4 concentrations are lower, the unbound or free fraction of circulating T4 is higher, and hormone metabolism is more rapid in most domestic animals than in humans (Bigler, 1976; Kaptein et al., 1994; Larsson, 1985; Larsson et al., 1985). The free hormone hypothesis, proposed by Robbins and Rall six decades ago (1960) and restated by Mendel (1989), states that it is the unbound fraction of hormone that is available to tissues and therefore proportional to the action, metabolism, and elimination of that hormone. This hypothesis has stood the clinical test of time; direct or indirect measurements of free T4 have been a mainstay in the diagnosis of thyroid disease in human and veterinary medicine. There is also strong evidence that certain cell types actively transport or exchange thyroid hormone from the plasma into the cytosol. The presence of a plasma membrane protein specific for thyroid hormone transport (see Section Plasma Membrane Transporters and Cytosolic Binding Proteins) certainly reflects the premium the cell is willing to pay to facilitate entry and possibly concentrate thyroid hormone. However, the serum binding proteins, particularly albumin and TTR, also serve the role of distributing the hormones to specific tissues. TTR carries both thyroid hormones and retinoic acid in the circulation. Most theories of thyroid hormone exchange have assigned a passive “reservoir” role to cytosolic thyroid hormone-binding proteins (CTBP; CBP in Figure 28.4), the proteins that retain thyroid hormone in a predominantly bound state inside the cell. Little is known about the regulation of these proteins, which are often called “intracellular albumin” for their low specificity, low affinity, and high capacity. However, in renal cytosol, the affinity of CTBP may be acutely regulated by cellular redox potential, increasing when NADPH levels are high. CTBP has been shown to be identical to the protein µ-crystallin (Burrow et al., 1989; Hashizume et al., 1987; Kaptein et al., 1994; Mendel, 1989; Pardridge, 1981; Suzuki et al., 2007). Irrespective of the mechanisms, the following observations in the clinical patient must be recognized: The inverse correlation between the serum free T4 concentration and the cellular distribution volume of T4, which exists in all subjects regardless of thyroid state. In the healthy euthyroid dog or cat, about 0.1% of total concentration of serum T4 is free (i.e., not bound to thyroid hormone-binding proteins), whereas about 1% of circulating T3 is free (Ferguson and Peterson, 1992; Kaptein et al., 1994). The proportion of free hormone may change in response to drug administration or illness. For example, in obese cats, increased nonesterified free fatty acids compete for hormone binding, resulting in an increase in free serum thyroid hormone concentrations, and may have a similar effect to compete with cellular binding, leading to a form of thyroid hormone resistance to negative feedback manifested by an increase in total and/or free T4 concentrations. However, it appears that thyroid status of the animal does not change, since the absolute level of the free hormone concentrations tends to soon return to within normal range or remains relatively constant (Ferguson, 1988, 1989b, 1994; Ferguson et al., 2007). Most evidence suggests that the thyroid hormone uptake by tissues is proportional to, but not limited to, the free or unbound fraction of circulating hormone. Approximately 50 to 60% of the body’s T4 and 90 to 95% of the body’s T3 is located in the intracellular compartment (Fish et al., 1987). Certain organs, particularly the liver and kidney, can concentrate thyroid hormones and exchange hormone rapidly with the plasma. In humans, about 60% of the intracellular T4 is in these rapidly equilibrating tissues (liver and kidney), whereas only 6% of the intracellular T3 is in these tissues. About 80% of all extrathyroidal T3 is located in the slowly equilibrating tissues (e.g., muscle, skin), while only 20% of intracellular T4 is in this compartment. As a result, most of the body’s T4 is located in plasma, interstitial fluid, liver, and kidney. The majority of the body’s extrathyroidal T3 is in the cells of the muscle and skin and in a conjugated form in the intestinal tract. Kaptein et al. (1994) have used a three-pool model to describe the distribution and metabolism of thyroid hormone metabolites and iodide (Figure 28.6). Table 28.1 compares the model parameters for T4, T3, rT3, and iodide in dogs, cats, and humans. Figure 28.6 Compartmental model of thyroid hormone metabolism. Table 28.1 Thyroid hormone and iodide kinetics in normal dogs, cats, and humans using a three-pool model. Source: Kaptein et al., 1994. Dog and human data from Kaptein et al., 1993. Cat data from Hays et al., 1988. Parameter values were estimated using a three-pool model. Only mean values are presented. Conversion to SI units: Total T4 in µg/dL × 12.87 = nmol/L; total T3 and reverse T3 in ng/dL × 0.01536 = nmol/L. aThyroid gland unblocked for all species. bDog data from Belshaw et al., 1974. cData from Hays and Solomon, 1965 (reanalyzed in three-pool model); Belshaw et al., 1974. As a principle impacting the diagnosis and treatment of thyroidal diseases, the tissue-specific regulation of deiodinases and TR receptor subtypes (see Section Correlation of Clinical Effects with Cellular Actions) suggests that the determination of thyroid status really must be established on a tissue-by-tissue basis. The plasma half-life of T4 in the dog has been estimated to be 8 hours (Kaptein et al., 1993, 1994), 10–16 hours (Fox and Nachreiner, 1981), and 11.6 hours (Le Traon, 2008), and 11 hours in the cat (Kaptein et al., 1994), compared to a plasma half-life of about 7 days in humans. Similarly, the plasma half-life of T3 in the dog has been estimated to be 5–6 hours, compared to 24–36 hours in humans (Table 28.2). Studies in the normal cat indicate that the plasma half-lives of T4 and T3 are similar to that of the dog (Fox and Nachreiner, 1981; Kaptein et al., 1994). Despite these rates of plasma clearance, the suppressive effect on pituitary TSH secretion appears to persist at least 24 hours after a dose, suggesting that intrapituitary T3 persists longer than the serum half-life (Ferguson, 2007; Le Traon, 2009). Therefore, as with many lipophilic nuclear receptor–mediated hormones, the plasma disappearance rates may underestimate the extent or duration of biological action. Table 28.2 Thyroxine kinetics in dogs, cats, and humans following intravenous, subcutaneous, and oral L-thyroxine administration. Source: Kaptein et al., 1994. Data on dogs and humans from Kaptein et al., 1993. Conversion to SI units: Total T4 in µg × 1.287 = nmol. aData from Hays et al., 1988. bData from Hays et al., 1992. cData from Hulter et al., 1984. In humans, and increasingly recognized in domestic animals, a wide range of clinical conditions, such as chronic starvation or malnutrition, surgery, diabetes mellitus, hepatic and renal disease, and chronic systemic illness, may result in decreased serum T3 concentrations together with elevated serum rT3 values (Araujo and Carvalho, 2011). This “low T3” syndrome results from inhibition of 5′-deiodinase, the enzyme necessary for conversion of T4 to T3 and the conversion of rT3 to 3,3′-T2 (Braverman and Utiger, 1991; Burrow et al., 1989; Kaptein et al., 1994; Kaptein, 1986; Daminet and Ferguson, 2003). The reduction in the production of T3, the most potent thyroid hormone, appears to be a beneficial adaptive mechanism by which the body serves to limit the loss of protein and perhaps blunt the metabolic rate during illness. Maintenance of serum T3 concentrations (with triiodothyronine replacement therapy) in euthyroid fasting humans leads to excessive nitrogen excretion and blunting of the pituitary’s TSH response to TRH, as if there was a state of hyperthyroidism. The body of evidence does not support the contention that lowering of serum T3 during malnutrition and illness is associated with “tissue” hypothyroidism. Also, outside of these regulatory mechanisms, there is, as yet, no evidence that a congenital or acquired 5′-deiodinase deficiency exists in animals or in specific tissues (Braverman and Utiger, 1991; Ferguson, 1984, 1988; Kaptein et al., 1993, 1994; Kaptein, 1986). In acute and severe illnesses in humans, serum T4 and T3 concentrations may also fall, in what is called the “low T4 state of medical illness.” Impaired serum protein binding of T4 caused by inhibitors of binding (such as free fatty acids) or a reduction in binding protein concentration results in reduced total serum T4 concentrations and increased free fractions of T4. In most cases, however, the absolute free T4 concentrations remain normal. A fall in serum TSH concentrations may also contribute to the subnormal serum T4 concentrations, especially in human patients treated with dopamine or glucocorticoids, drugs inhibiting TSH release. No studies have examined systematically the benefit or detriment of thyroid hormone therapy in domestic animals; however, studies of critically ill patients with low serum T4 and T3 concentrations have revealed that thyroxine therapy is not beneficial and fails to improve survival (Kaptein, 1986; Kaptein et al., 1993, 1994). The effects of nonthyroidal illness on thyroid hormone metabolism in the dog are less well characterized than in humans. In the dog, depressed serum T4 concentrations have been reported in various nonthyroidal illnesses such as hyperadrenocorticism (e.g., Cushing’s syndrome), diabetes mellitus, hypoadrenocorticism (e.g., Addison’s disease), chronic renal failure, hepatic disease, as well as a variety of other critical medical illnesses requiring intensive care (Ferguson, 1984, 1988, 1994, 2007; Ferguson and Peterson, 1992). This topic was reviewed by Daminet and Ferguson in 2003. A variety of drugs may impair plasma or tissue binding of the thyroid hormones or alter thyroid hormone metabolism. Drugs used in veterinary medicine that are most likely to alter circulating thyroid hormone concentrations include the glucocorticoids, anticonvulsants, quinidine, salicylates, phenylbutazone, and radiocontrast agents. The mechanisms by which these drugs exert their effect vary. Quinidine and other membrane-stabilizing drugs may inhibit 5′-deiodinase. Salicylates, furosemide, and oleic acid may directly displace thyroid hormone from plasma binding sites (Daminet and Ferguson, 2003; Ferguson, 2007; Ferguson et al., 2007). Phenylbutazone appears also to have a direct antithyroid (goitrogenic) effect in some species, having been shown to decrease total and free T4 in the horse (Ramirez et al., 1997). In vitro, it appears to decrease serum hormone binding. Radiocontrast agents (e.g., diatrizoate, iopanoic acid, ipodate, tyropanoate, and metrizamide) may act by preventing the uptake of T4 by tissue, by directly inhibiting 5′-deiodinase, or by releasing the iodine they contain to exert an antithyroid effect on the thyroid gland. No studies have been reported in domestic animals to evaluate the influence of these iodine-containing drugs on thyroid function tests or on subsequent radioiodine uptake (Ferguson, 1984, 1989b, 1994). Exogenous glucocorticoids have also been shown to have a profound effect on thyroid function tests in the dog, but similar studies have had little effect on serum T4 levels in the cat. A single high immunosuppressive dosage of glucocorticoid (2.2 mg/kg prednisone, 0.6 mg/kg dexamethasone) will lower serum T3 but not serum T4 concentrations in the dog (Kemppainen et al., 1983; Laurberg and Boye, 1984). Serum T3 concentrations may be decreased because of glucocorticoid inhibition of 5′-deiodinase or simply because of a reduced availability of plasma T4, the substrate for the enzyme. Most dogs on chronic, high-dose, daily glucocorticoid therapy will have very low or undetectable serum T4 concentrations, as well as subnormal serum T3 values (Ferguson and Peterson, 1992; Kaptein et al., 1992; Kemppainen et al., 1983; Moore et al., 1993; Torres et al., 1991). Based upon electron microscopic examination of thyroid tissue, it was postulated that glucocorticoids may interfere with thyroid hormone secretion by inhibiting lysosomal hydrolysis of colloid in the follicular cell (Woltz et al., 1983). Immunosuppressive dosages (1.1–2 mg/kg q 12 h) of prednisolone in dogs for 3 to 4 weeks significantly decreased serum total T4 and to a lesser extent, FT4 concentrations. These changes were observed as soon as 1 day after initiation of treatment (Torres et al., 1991; Daminet et al., 1999). Endogenous TSH was not affected by 3 weeks of an immunosuppressive dosage of prednisone, but the assay may not allow detection of a mild decrease. No changes in serum total T4 levels were seen after a month of prednisone administration at an antiinflammatory dosage (0.5 mg/kg q 12 h) (Moore et al., 1993). However, a decrease in total T3 concentrations with a supranormal T4 increase after TSH administration was observed. Another study also showed that prednisone at an antiinflammatory dosage of 1 mg/kg/day had no effect on TSH concentration of dogs with experimental hypothyroidism, but higher dosages have not been studied. A subsequent study in T4-supplemented hypothyroid dogs showed that prednisone at a dosage of 1 mg/kg PO q 24 h for 7 days decreased total T4, while FT4 was unchanged, suggesting that FT4 may be less affected by daily prednisone administration. Subsequent antiinflammatory doses of prednisone administered every other day did not alter T4 or TSH concentrations (O’Neill et al., 2010). In summary, glucocorticoids can markedly decrease total T3 and T4 concentrations and to a lesser extent FT4 concentrations in dogs. Therefore, thyroid function test results should be interpreted carefully in any dog receiving glucocorticoids if prescribed an immunosuppressive dosage or for a period greater than 1 month (Ferguson, 1984, 1994; Moore et al., 1993; Daminet and Ferguson, 2003). In the horse, 50 g dexamethasone q 12 h topically for 10 days led to a 30–40% decrease in both T3 and T4 with nadir at day 2 for serum T3 concentration and day 6 for serum T4 concentration. Neither hormone concentration had recovered by 11 days after discontinuation of therapy (Abraham et al., 2011). Other drugs have been well documented to alter thyroid hormone metabolism or serum or tissue binding of the thyroid hormones in the dog. The anticonvulsants diphenylhydantoin and phenobarbital, which are mixed function oxidase inducers, consistently decrease serum T4 concentrations. Phenobarbital increases the rate of clearance of T4. Increased hepatic deiodination of thyroid hormones, biliary clearance, and fecal excretion result in decreased concentrations of circulating thyroid hormones. The short-term administration (3 weeks) of phenobarbital to dogs did not affect total or free T4 nor TSH serum concentrations in beagle dogs. However, several studies have looked at the long-term effects of phenobarbital administration. Total and free T4 can be decreased to a range consistent with hypothyroidism. Endogenous TSH concentrations can remain within reference range or be slightly increased. Serum phenobarbital concentrations did correlate with the decrease in total and free T4 concentrations in one study, but not in another. The reduction of free T4 concentration particularly supports the idea that phenobarbital influences the steady-state clearance of T4. Difficulty of interpretation of drug effects is compounded by the observation in dogs that seizure activity reduces serum total T4 concentrations in proportion to the frequency of seizure episodes (von Klopmann et al., 2006). In one study, thyroid function normalized 1 to 4 weeks after discontinuation of phenobarbital. It is therefore recommended, when this is an option clinically, to evaluate thyroid function at least 4–6 weeks after discontinuation of phenobarbital (Kantrowitz et al., 1999; Gaskill et al., 1999; Müller et al., 2000; Gieger et al., 2000; Daminet et al., 1999; McClain et al., 1989; Curran and DeGroot, 1991; Johnson et al., 1993; Barter and Klaassen, 1994; Theodoropoulos and Zolman, 1989; Liu et al., 1995; DeSandro et al., 1991; Attia and Aref, 1991). The antiepileptic potassium bromide is a halide chemically related to iodide and could potentially interact with iodine in the thyroid gland. In rats, bromide produces a relative iodine deficiency, thereby interfering with iodine uptake, iodine transport, and iodination of tyrosine and tyrosyl residues on thyroglobulin. In one study of epileptic dogs and another of healthy dogs receiving KBr at therapeutic dosages up to 6 months, no abnormal thyroid function test results were seen (Kantrowitz et al., 1999, Paull et al., 2000). A study evaluated the effect of a standard dosage of trimethoprim/ sulfamethoxazole on thyroid function tests in dogs with pyoderma and normal baseline serum thyroxine concentrations. The average serum T4, but not T3, concentration fell significantly during the treatment period of 6 weeks. The TSH response also fell in several dogs and radionuclide imaging suggested that the preparation (likely the sulfa component) interfered with iodine metabolism by the thyroid gland. The thyroid carcinogenic potential of sulfonamides, which has resulted in restriction of some forms in food animals, is likely due to its goitrogenic potential, chronic elevation of serum TSH, and subsequent stimulation of thyroid growth (Hall et al., 1993). Sulfonamides are known goitrogens in domestic species because they can markedly interfere with thyroid hormone synthesis through reversible inhibition of TPO activity and reduction of serum concentrations of thyroid hormones. With reduced negative feedback, there is an increase in pituitary secretion of TSH, which induces proliferative changes in the thyroid gland. Long-term administration of sulfonamides and prolonged stimulation of the thyroid gland by TSH have been associated with thyroid neoplasia in rats. There is considerable interspecies variation with respect to TPO inhibition by sulfonamides. For example, only mild effects of sulfonamides are observed on human thyroid function. Several prospective studies have evaluated the effects of sulfonamide administration on canine thyroid function. Trimethoprim-sulfadiazine administered at a dosage of 15 mg/kg q 12 h to healthy dogs for 4 weeks had no effect on serum total T4 or T3 or free T4 concentrations or upon the results of TSH stimulation tests. When trimethoprim-sulfadimethoxazole was administered to dogs with pyoderma for 6 weeks at a higher dosage of 30 mg/kg q 12 h, serum total T4 and T3, and FT4 concentrations decreased. Half of the dogs in this experiment had total thyroxine (TT4) levels below the reference range at the end of the treatment, which could easily have resulted in the inappropriate diagnosis of primary thyroid failure. In another study, the same dosage of trimethoprim-sulfadimethoxazole was given to healthy dogs for 6 weeks, and thyroid hormones were markedly decreased and endogenous TSH increased as soon as 7 days after initiation of the treatment. Thyroid imaging showed increased uptake of pertechnetate, and thyroid gland biopsies revealed hyperplasia of thyroid follicles and absence of colloid production. These findings further support the concept that sulfonamides act as goitrogens, resulting in primary hypothyroidism with secondary changes associated with the proliferative effects of TSH. The effects of sulfonamides on thyroid function are species specific, and dosage and duration dependent. Normalization of thyroid function test results in dogs after cessation of sulfonamide administration can take up to 8 or 12 weeks. Sulfa drugs can lead to clinical hypothyroidism and even goiter in some dogs (Cohen et al., 1980, 1981; Lagler et al., 1976; Panciera and Post, 1992; Post et al., 1993; Hall et al., 1993; Campbell et al., 1996; Gookin et al., 1999; Taeymans and O’Marra, 2009). Many nonsteroidal antiinflammatory drugs (NSAIDs) have been shown to alter thyroid function tests in humans, since circulating thyroid hormones are highly protein bound, and various NSAIDs can displace thyroid hormones from serum protein-binding sites. Short-term administration of therapeutic dosages of salicylates leads to a transient increase in unbound hormone levels and suppression of TSH concentrations. After long-term treatment with salicylates, a new steady-state is reached, reflecting an increased T4 turnover rate, and serum total T4 concentrations have been reported to be reduced 20–40%. Free T4 concentrations can be unchanged or decreased, and TSH concentrations return to the reference range within a few weeks of treatment. However, a 60-day double-blind study of osteoarthritic euthyroid dogs given placebo, meloxicam, carprofen, or chondroitin sulfate/glucosamine identified no drug-associated changes in serum total and free T4 concentrations or TSH (Sauve et al., 2003). Thyroid hormone (T4 and/or T3) acts on many different cellular processes via specific ligand–receptor interactions with the nucleus, the mitochondria, and the plasma membrane (Figure 28.4). The effects of thyroid hormone are seen in most tissues throughout the body. Although both L-T4 and L-T3 have intrinsic metabolic activity, L-T3 is three to ten times more potent in binding to the nuclear receptors and similarly more potent in stimulating oxygen consumption (Table 28.3). Except for the deaminated form of T4 and T3 (tetraiodothyroacetic acid (Tetrac) and triiodothyroacetic acid (Triac), respectively),x most thyroid hormone metabolites have little thyromimetic activity. Reverse T3 (3,3′,5′-T3) has recently been linked to some potential developmental effects in the central nervous system. Table 28.3 Relative nuclear binding affinity of thyroid hormone analogs to T3. Source: Oppenheimer, 1983. Reproduced with permission of Elsevier. The effects of thyroid hormone can generally be divided into those that are rapid and evident within minutes to hours of administration, such as stimulation of amino acid transport and mitochondrial oxygen cons1981umption, and those that require protein synthesis and a longer period of time (usually no sooner than 6 hours) to be manifested. Of course, the clinical manifestations may require weeks to months to clearly appreciate. About one-half of the increment in oxygen consumption produced by thyroid hormone has been related to activation of the plasma membrane-bound Na+,K+-ATPase, which, at least in the kidney and liver, is secondary to increases in passive K+
Thyroid Hormone and Antithyroid Drugs
Introduction
Hypothyroidism
Hyperthyroidism
Thyroid Physiology
Iodine Metabolism
Thyroid Hormone Synthesis
Thyroid Hormone Secretion
Hypothalamic–Pituitary–Thyroid Extrathyroid Axis
Negative Feedback Regulation
Metabolism of Thyroid Hormone
Types and Regulation of Deiodinase Enzymes
(B) Functional domains of a nuclear thyroid hormone receptor. The functional domains of the thyroid hormone receptor are similar among the four active isoforms (α1, β1, β2, and β3), with the only difference being in the amino-terminal section. Coactivators and corepressors generally interact with the T3 binding domain, which also dimerizes with the retinoic acid receptor (RXR).
Plasma Hormone Binding of Thyroid Hormone
Tissue Thyroid Hormone Uptake: the “Free Hormone” Hypothesis
Dogs
Cats
Humans
T4
Plasma concentration (ng/dL)
2.8
1.68
6.8
Free fraction (%)
0.102
0.056
0.030
Total mean residence time (d)
0.54
0.69
6.8
Clearance rate (L/[kg·d])
0.24
0.38
0.017
Degradation rate (µg/[kg·d])
6.81
6.2
1.14
Total pool size (µg/kg)
3.7
4.3
7.8
Distribution (%)
Plasma pool
46
13
32
Rapidly equilibrating pool
23
19
26
Slowly equilibrating pool
31
68
42
T3
Plasma concentration (ng/dL)
43
32
140
Free fraction (%)
1.426
0.48
0.299
Total mean residence time (d)
0.40
0.62
1.50
Clearance rate (L/[kg·d])
1.99
1.77
0.32
Degradation rate (µg/[kg·d])
0.84–0.89
0.52–2.2
0.43–0.46
Total pool size (µg/kg)
0.34
0.30
0.66
Distribution (%)
Plasma pool
7
3.6
9
Rapidly equilibrating pool
29
18
19
Slowly equilibrating pool
64
79
73
Reverse T3
Plasma concentration (ng/dL)
22
—
13
Free fraction (%)
0.560
—
0.111
Total mean residence time (d)
0.12
—
0.16
Clearance rate (L/[kg·d])
1.44
—
1.78
Degradation rate (µg/[kg·d])
0.32–0.42
—
0.23–0.33
Total pool size (µg/kg)
0.039
—
0.036
Distribution (%)
Plasma pool
31
—
15
Rapidly equilibrating pool
29
—
29
Slowly equilibrating pool
40
—
55
Inorganic iodidea
Plasma mass (µg/kg)b
21
—
1.67
Total mean residence time (d)
1.07
0.82
0.35–0.36c
Clearance rate (L/[kg·d])
0.43
0.63
1.00–1.01
Total distribution volume (L/kg)
0.46
0.44
0.35–0.36
Distribution (%)
Plasma pool
19
11
13c
Rapidly equilibrating pool
9
32
38
Slowly equilibrating pool
72
57
50
Metabolic Clearance Rates
Dogs
Cats
Humans
T4 degradation rate (µg/[kg·day])
6.81
6.2
1.14
Intravenous
Time to peak (hour)
0.02–0.03
0.02–0.03a
0.02–0.03
Total mean residence time (hour)
13.0
16.6a
164
Terminal half-life (h)
7.6
10.7b
168
Subcutaneous
Dosage (µg/[kg·day])
5–7
—
—
Absorption (%)
100c
—
81
Time to peak (h)
1.5–1.8
—
72
Terminal half-life (h)
14.7
—
120
Oral
Dosage (µg/[kg·day])
20–40c
20–30c
2.11
Absorption (%)
10–50c
10.5c
50–80
Time to peak (h)
—
3–4c
2–4
Terminal half-life (h)
7.6
10.7c
168
Extrathyroidal Factors Altering Thyroid Hormone Metabolism
Effect of Illness and Malnutrition in Humans
Effect of Drugs on Thyroid Function
Mechanisms of Thyroid Hormone Action
Correlation of Clinical Effects with Cellular Actions
Relative binding affinity (T3 = 1)
Analog
In vitro
In vivo
L-T3
1.0
1.0
D-T3
0.6
0.7
Triiodothyroacetic acid (triac)
1.6
1.0
Isopropyl T2
1.0
1.0
L-T4
0.1
0.1
Tetraiodothyroacetic acid (Tetrac)
0.16
0.05
3,3′,5′-T3 (reverse T3)
0.001
0
Monoiodotyrosine
0
0
Diiodotyrosine
0
0
Stay updated, free articles. Join our Telegram channel
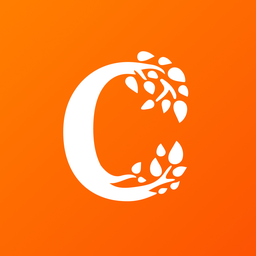
Full access? Get Clinical Tree
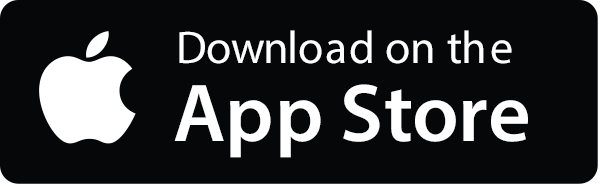
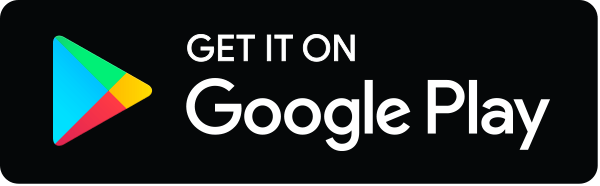