Chapter 8 To deliver maximal performance it is essential that a horse has good balance. A part of the required balance comes from factors connected to the hoof (Balch et al., 1991a; Curtis, 1999, Aoki, 1999; Arabian et al., 2001; Eliashar, 2007). ‘No foot, no horse’ is a well-known saying that emphasizes the important role of the hoof (Davies, 2002). After domestication of the horse 5000–6000 years ago, man assumed much of the responsibility for the balance between hoof growth and hoof wear. The Greek horse people (hippiaters) were in favor of breeding horses with good hoof quality that did not need shoes. The Romans invented the hipposandal that was attached to the hoof with straps and was used to protect the feet of the horse en route to the battlefield, where they were removed. The first iron horseshoes with nails were made by the Celts 2000 years ago and were similar to the ones we use today (Fig. 8.1). Fig 8.1 The development of the horseshoe. (A) Ancient grass sandal. (B) Roman iron hipposandal fastened without nails. (C) Celtic horseshoe with oval nailholes. (D) Medieval shoe with square nail holes. (E) Renaissance shoe with fullering. Reprinted with kind permission of Butler, Doug, The Principles of Horseshoeing, 1995, Doug Butler Enterprises, Inc. www.dougbutler.com. During locomotion every stride involves forces at the hoof–ground interface that load the locomotor apparatus. Repeated application of forces that have a high magnitude and/or an abnormal direction of action, overload the limb and may lead to the development of pathological processes (Johnston & Back, 2006). When we have an insight how these forces work, we can modulate them by corrective hoof trimming and optimal shoeing as rational measures to treat and prevent lameness, e.g. at purchase (Anderson, 1992). That is the basic theme of this chapter. The equine foot has evolved from the third digit, which has been greatly elongated and strengthened. The hoof wall has developed from the nail of the third digit. The hoof of the first digit is still present, though rudimentary, in the form of the chestnut on the medial side of the radius (forelimb) or metatarsus (hind limb), while that of the fifth digit, especially in Coldbloods, persists as the ergot on the palmar/plantar side of the metacarpo-/metatarsophalangeal joint (Dyce & Wensing 1980; Pollitt 1995). The hoof capsule consists of several parts: the coronet, the wall, the sole, the frog and the heels (Fig. 8.2). The horn of each part of the hoof is produced by a corresponding area of dermis (corium). The estimated horn regeneration time based on a growth rate of 8–10 mm/month is 12 months for the toe, 6–8 months for the quarters, and 4–5 months for the heels. The coronet separates and connects the skin above from the hoof wall below. Deep to the coronet lies the dermis that produces the horn tubules of the hoof wall via the horn papillae, which project distally into the horn of the hoof wall. The horn tubules are responsible for the striated appearance of the hoof wall. The nonpigmented innermost part of the tubular hoof wall is less stiff than the outer part and serves as a mechanical buffer zone assisting the feet of horses in transmitting load through the tissues without inflicting damage (Wagner et al., 2001, 2002). Projecting from the inner surface of the hoof wall are the primary epidermal lamellae (600/hoof) and secondary epidermal lamellae (100–200/primary lamella). The insensitive horn of these epidermal lamellae interdigitates with the sensitive dermal lamellae to form the functional connection between the hoof wall and P3. The horn that forms the epidermal lamellae is unstructured (non-tubular). As the hoof wall grows, it moves distally by a mechanism that allows the primary epidermal lamellae to slide past the stationary secondary epidermal lamellae. Primary epidermal laminae morphology (spacing, orientation and curvature) is responsive to mechanical stress at the laminar junction (Thomason et al., 2005, 2008). On the solar surface, the white line demarcates the junction between sensitive and insensitive tissues and is in fact a distal projection of dermal and epidermal laminae (cf. terminal papillae). When shoes are nailed in place, the nails should enter peripheral to the white line. The bony skeleton of the hoof consists of the distal phalanx (P3) or coffin bone, the navicular bone and part of the middle phalanx (P2) or short pastern bone. All three of these bones take part in the formation of the distal interphalangeal or coffin joint (Fig. 8.3). During flexion of the coffin joint, the navicular bone generally follows the coffin bone although small but consistent motions exist between the navicular bone and the coffin bone (Bowker et al., 2001; Van Dixhoorn et al., 2002). The medial and lateral hoof cartilages (ungual cartilages) are square-shaped structures located on either side of P3 and are connected to P1 and P2 by connective tissue. They are large and flexible. Between them lie the digital cushion and a venous plexus. Compression of these structures during locomotion assists the return of venous blood to the heart (Hoffman et al., 2001; Pietra et al., 2004). The navicular bone is connected to P3 by the distal navicular or ‘impar’ ligament, to P2 by connective tissue and the synovial membranes of the coffin joint, navicular bursa and tendon sheath, and to P1 by the proximal navicular ligaments. The navicular bone is covered on one side by hyaline cartilage and on the opposite side by fibrocartilage (Viitanen et al., 2003b). Its hyaline cartilage side is in contact with the palmar/plantar aspect of P2 and P3. The deep digital flexor tendon (DDFT) curves around the palmar/plantar aspect of the navicular bone before attaching to the flexor surface of P3. The navicular bursa is interposed between the DDFT and the fibrocartilage of the navicular bone. The blood supply enters the hoof via the palmar/plantar digital arteries, which run through the terminal arch within P3 (Fig. 8.4). A network of arteries perforates the dorsal surface of P3 and ramifies in the lamellar dermis. Some branches from this plexus are directed proximally to supply the coronet, where they anastomose with branches of the circumflex artery of the coronet. The dorsal branches leave the palmar/plantar digital arteries just before they enter the terminal arch of P3. The palmar/plantar branches run to the heel region where they form the venous plexus and anastomose with the circumflex artery of the sole. The hoof is innervated by the palmar/plantar digital nerves, which are located caudal to the vein and artery in the pastern region (Fig. 8.5). The nerves can be blocked by injection of a local anesthetic at the proximal pastern level where the nerves are crossed by the ligament of the ergot (low palmar block) or more distally as the nerve passes deep to the hoof cartilage (palmar digital block). Palmar digital nerve blocks anesthetize the caudal third of the hoof and its contents; low palmar nerve blocks just distal to the fetlock joint anesthetize the entire hoof. Bowker et al. (1993, 1995) described the concentration of nociceptors in the palmar/plantar part of the frog and in the proximal navicular area. It is hypothesized that these so-called lamellar bodies supply the central nervous system and the brain with proprioceptive information describing the location of the body in space (Van Wulfen & Bowker, 2002). This information is needed to control the central pattern generator (CPG) and thus intra- and inter-limb coordination (Fig. 8.6). Fig 8.6 (A) Sensory receptors in the foot. In the sagittal view the four zones of the sole (S1–S4) represent locations of the lamellated corpuscles with the relative density being indicated by the intensity of the green shading in zones S1–S4. S4 shows the highest density of lamellated corpuscles. In the ventral view the lamellated corpuscles were obtained from the areas shaded in green. (B) Sensory receptors in the navicular region. Inset shows the three levels from which drawings a–c were obtained. Note that the lamellated corpuscles (dots) are located primarily abaxially and proximal to the collateral sesamoidean ligament (CSL) often in association with the palmar digital nerve (n), as well as with the artery (a) and vein (v). d is a parasagittal drawing from the abaxial region of CSL. Sensory receptors are shown as red dots. PII: pastern bone; PIII: coffin bone; DDF: deep digital flexor tendon; Nav: navicular bone. (A) Reprinted from Bowker, 1993, Sensory receptors in the equine foot, American Journal of Veterinary Research, with permission from American Journal of Veterinary Research http://avmajournals.avma.org/loi/ajvr. (B) Reprinted from Bowker, R.M., Linder, K.E., Sonea, I.M. and Guida, L.A. (1995) Sensory nerve fibers and receptors in the equine distal forelimbs and their potential role in locomotion. Equine Vet. J. 18, 141–146, with permission of the Equine Veterinary Journal. Studies of the effects of local anesthesia in sound horses have yielded conflicting results. Keg et al. (1996) demonstrated no effect of a low palmar nerve block on gait symmetry as evaluated kinetically, whereas Kübber et al. (1994) did detect some kinematic effects. It is not known whether the differences between these studies were due to the sensitivity of the analytic equipment or to the (un-) soundness of the horses. Ideally, hoof trimming optimizes the interaction between the hoof and the ground during locomotion. Since the hoof is a three-dimensional structure, it should be balanced in both the craniocaudal and mediolateral planes. Forces at the toe, medial and lateral heels collectively are optimally distributed when the hoof and pastern angles are aligned (Balch et al., 1997; Davies, 2002). Craniocaudal balance evaluates the hoof in a lateral view (Fig. 8.7). It is assessed with the horse standing square on a level surface. Alignment of the dorsal hoof wall with the pastern axis is achieved by adjusting the absolute and relative lengths of the heels, the quarters and the toe. A hoof that is balanced in this manner usually contacts the ground flat-footed or slightly heel first. When the hoof has a more acute angle than the pastern, the hoof–pastern axis is said to be broken backwards. Conversely, when the angle of the hoof is more upright than that of the pastern, the hoof–pastern axis is said to be broken forward. Radiographic studies have shown that P1 is always a little more upright (vertical) than P2 and P3 with the three phalanges being most closely aligned when the hoof is trimmed with the dorsal hoof wall parallel to the pastern axis (Bushe et al., 1987). Under these circumstances the angle between the solar surface of P3 and the ground is approximately 5°, whereas the dorsal face of P3 is parallel to the dorsal hoof wall at a distance of about 19 ± 0.5 mm in Warmbloods (Back, 2001). Fig 8.7 Diagrams of straight, ideal and correct hoof–pastern axes and common deviations viewed (A) from the front and (B) from the side. Reprinted with kind permission of Butler, Doug, The Principles of Horseshoeing, 1995, Doug Butler Enterprises, Inc. www.dougbutler.com. There is a difference between the shape of the fore and hind hooves: the fore hoof is wider and usually has a smaller (more acute) hoof angle, while the hind hoof is narrower and has a larger hoof angle. In farriery books (Hermans, 1984; Hertsch et al., 1996; Ruthe et al., 1997), angles ranging from 45–50° for the forelimbs and 50–55°for the hind limbs have been reported, but more recently the average angles have been reported to be over 50° in the forelimb and over 55° in the hind limbs (Clayton, 1988; Kobluk et al., 1990; Balch et al., 1991a; Butler, 1995; Hickman & Humphrey, 1997; Oosterlinck et al., 2010c). In racehorses, a significant decrease in hoof angle was observed in association with starting fast exercise work (Peel et al., 2006). According to the same study, wet pasture conditions may also be associated with a reduced hoof angle. There exist also differences in hoof shape between contralateral limbs. A study on radiographic measurements of the front feet of normal Warmblood horses found that in 70% of the horses the left hoof capsule and coffin bone were significantly larger than the right (Kummer et al., 2006; White et al., 2008). According to Wilson et al. (2009), contralateral differences in hoof spread are related to generalized asymmetry in forelimb left–right morphometry. Contralateral differences in hoof shape may suggest unequal loading of limbs, which in turn may contribute to injuries and reduced performance. Kroekenstoel et al. (2006) determined that foals with uneven feet show asymmetrical loading of the interphalangeal joints (Lejeune et al., 2006), whereas foals are born with a broken back-foot axis that becomes more upright with increased bone growth and tendon strength. The second component of craniocaudal balance is the location of the bearing surface of the hoof relative to the weight-bearing axis through the cannon bone. The bulbs of the heel should lie vertically below the central axis of the cannon bone in the sagittal plane. In some horses, although the hoof–pastern axis is aligned, the whole hoof capsule is located too far forward so that the bulbs of the heels are ahead of the central axis of the cannon bone. The resulting caudal concentration of the weight bends the hoof tubules at the heels, which reduces their ability to withstand compression, and leads to underrun heels (Stashak, 1987; Balch et al., 1997). Normally, the dorsal toe wall and caudal heel wall should run parallel to each other and the toe wall length relative to heel wall length should be 2 : 1.0 in the fore hooves and 2 : 1.5 in the hind hooves (Hermans, 1984). Underrun heels have been defined as having a difference between the angles of the toe and heels that is more than 5° (Balch et al., 1997). Mediolateral balance evaluates the hoof in a frontal plane and attempts to optimize hoof balance by either a static or a dynamic evaluation (Seeherman, 1991; Balch et al., 1997). Static balance seeks to achieve symmetry in the square standing horse so that a line that bisects the limb longitudinally is intersected at 90° by a transverse line drawn across the heels (geometric limb axis). Caudron et al. (1997a,b, 1998) used radiographs to evaluate and correct this balance. In the dynamic method, the hoof is trimmed so that the medial and lateral sides contact the ground simultaneously, which adds a new dimension to the equation and makes the solution even more elusive. In a horse with ideal conformation, static and dynamic balancing will show a rather similar result, but when conformational defects are present the two methods produce different results. When a horse is standing quietly, the force due to gravity is compensated by the ground reaction force (GRF) acting near the geometric center of pressure (CP) of the foot, which lies around the apex of the frog (Barrey, 1990; Balch et al., 1997; Ovnicek, 1997). The hoof rotates around the instantaneous center of rotation of the coffin joint, which is located in the distal part of P2. Equilibrium exists when the moment (torque) of the GRF about the coffin joint (FGRF* dGRF) is equal to the moment of the DDFT (FDDFT* dDDFT). Ideally, the central reference point (CRP) should be located midway between the toe and the heels (Wright & Douglas, 1993), although in wild horses the distance from the toe to the CRP is only about one-third of the toe-to-heel distance (Ovnicek, 1997; Fig. 8.8). Ovnicek (1997) studied the hooves of 65 wild horses. From his observations he developed the natural trimming technique for unshod horses. The principles of this technique are that the heels are trimmed back to the widest point of the frog, along the sole plane. The toe is beveled to a 15–20° angle in a manner akin to what would be done in preparation for a rocker-toe shoe. The quarters are hollowed slightly by rasping so they are not weight-bearing on a firm surface. This leaves a raised area projecting downwards at the heel buttress. There is a gradual arc between the impression mark at the lateral side of the toe and the heel impression mark on each side of the foot. The hoof wall has four loading points: one at each side of the toe and one at each heel. Little to no sole, frog and bars are ever removed. Within a few months this method of trimming should result in a stronger hoof structure with a cupped sole, spreading heels, and a well-developed frog. Dejardin et al. (2001) demonstrated that four point trimming results in strain concentration above the hoof contact points with the strain magnitude being dependent on contact area. Hood et al. (2001a) found that on a deformable surface, load distribution is principally solar and located transversely across the central region of the foot. Therefore, it is hypothesized that the typical hoof conformation observed in feral horses is the result of increased erosion of the central solar and quarter regions of the bearing wall, allowing the heels and the lateral and medial toe to remain relatively long. The rapid disappearance of this initially noneroded areas when horses are maintained on a flat nondeformable surface further supports that they are caused by lack of contact when the horse is on a more natural deformable terrain. Hood et al. (2001a) concluded that so called four-point loading is the product of ground surface abrasion and, as such, is the mirror image of the loading pattern seen in horses kept on a natural terrain. Hence, the hoof ground interaction after an artificial four-point trim may only partially mimic the natural hoof ground interaction observed in feral horses. Several techniques have been used to document the kinematics and kinetics of the hoof. One of the requirements of the recording equipment is that it should have a high enough sampling frequency to distinguish events such as initial ground contact, heel-off and toe-off with sufficient accuracy (Linford, 1994). This can be especially difficult on outdoor tracks where reflective markers disappear in the sand (Merkens & Schamhardt, 1994; Scheffer & Back, 2001). When the horse is observed in the lateral view, initial ground contact is heel first, flat-footed or toe first. The hind limbs show a greater tendency to heel first contacts than the forelimbs, and heel first contacts occur more frequently during high-speed locomotion (Back et al., 1995). However, in some movements, such as piaffe, toe first contacts are normal. The frequency of toe first contacts increases when the hoof is trimmed with an acute angle (long toes and/or low heels). Conversely, when horses are trimmed with a steep hoof angle (short toe and/or long heels), heel first contacts are more numerous (Clayton, 1990a). Therefore, the manner of initial ground contact depends on speed, gait and farriery. Nevertheless, Van Heel et al. (2004) found that most horses tend to land on the lateral side of the foot, especially in the hind limbs, which is only minimally influenced by trimming. Immediately after initial ground contact, the hoof is rapidly decelerated by the vertical landing forces and horizontal braking forces that reduce its speed to zero during the impact phase that follows initial ground contact (Back et al. 1995; Burn 2006; Parsons & Wilson, 2006). The forces and decelerations associated with impact have been measured using a force plate (Merkens & Schamhardt, 1994; Merkens et al., 1994; Wilson & Pardoe, 2001; Weishaupt et al., 2002; Oosterlinck et al., 2010b), force shoe (Barrey, 1990; Frederick & Henderson, 1970; Ratzlaff et al., 1985, 1993; Kai et al., 2000; Rollot et al., 2004; Roland et al., 2005; Chateau et al., 2009a; Robin et al., 2009) and accelerometers (Benoit et al., 1993; Burn et al., 1997; Back et al., 2006). The hoof and interphalangeal joints attenuate the shock wave associated with impact (Dyhre-Poulsen et al., 1994; Lanovaz et al., 1998; Willemen et al., 1998). Approximately 67% of the damping of impact vibrations takes place at the interface between the hoof wall and the distal phalanx. The attenuation of impact vibrations at the interphalangeal joints and the metacarpophalangeal joint seems considerably less (12% and 9% respectively) leaving approximately 12% of the impact vibrations detectable at the level of the metacarpus (Willemen et al., 1999a). Nevertheless, the forces acting in different directions have the potential to damage the body (Fig. 8.9). Friction between the hoof and the ground and hardness of the ground affect the forces applied to the limb during the impact phase (Hjertén & Drevemo, 1993, 1994). The amplitude of impact vibrations at the level of the hoof wall is 15% higher in shod versus unshod hooves independent from the type of shoe that is used (shoes with or without pad). At the level of the first phalanx and the metacarpus the difference between shod and unshod vanishes (Willemen et al., 1999a). The impulsive loading that occurs during impact has been implicated as a causative factor in arthritis in animals (Radin et al., 1981; Pratt, 1997; Radin 1999) and in humans (Folman et al., 1986; Ker et al., 1989). The time during which these forces have to be absorbed is reduced as speed increases. For example, the time taken to absorb impact shock at the canter (7 m/s) is 50% of that at the walk (1.6 m/s), which is a consequence of the shorter time the foot is on the ground. Back et al. (1995) found that during trotting on a treadmill the hoof was flat on the ground, and the vertical speed and acceleration were zero within 3% (20 ms) of the total stride duration. Surprisingly, it took 6% (40 ms) of total stride duration for the horizontal speed to reach zero, but as demonstrated by Gustås et al. (2001) the time lapse of this horizontal retardation of the hoof is an important factor in the attenuation of the impact. The forelimbs appeared to land with a higher vertical velocity and the hind limbs with a higher horizontal velocity (Back et al., 1995). Thus, the forelimbs ‘bounce’, whereas the hind limbs ‘slide’. On the other hand, Gustås et al. (2004) found no significant difference in hoof deceleration between fore and hind limb using accelerometers mounted on the hooves. The sliding of the hoof in the sagittal plane has been measured for horses trotting on a treadmill; steel shoes slide for longer than rubber shoes and the hoof stops less abruptly on a coir mat than on a rubber belt (Roepstorff et al., 1994). It has been reported that the sliding of the hoof took 16 ms on a dirt track, whereas on a hard surface (asphalt or concrete) it took 21 ms for the unshod hoof and 32 ms for steel shoes (Back, 2001). Pardoe et al. (2001) could not demonstrate a significant difference in either slip time or distance between steel, plastic and rubber shoes, whereas Back et al. (2006) noted less gliding in freshly steel shod horses with protruding nails than in the same horses shod with plastic shoes. The active neurophysiological response time is the time required for the muscles to respond to a stimulus. It has been estimated to be around 30 ms in humans (Nigg et al., 1981) and horses (Bowker et al., 1993; Hjertén & Drevemo, 1993). This suggests that the forces acting on the equine lower limb during the impact phase are determined mainly by passive factors like coordination and ground properties (Hjertén & Drevemo, 1993). Since the hoof shows more elasticity in the heel area than at the toes or quarters, its geometry changes when it is loaded: the proximal dorsal wall moves back, the quarters flare to the side and sole and frog perform a downward movement (Hinterhofer et al., 2000, 2001; Burn & Brockington, 2001; Hobbs et al., 2004, 2009); this is called the hoof mechanism (Fig. 8.10). Fig 8.10 Schematic drawing of the hoof mechanism phenomenon. The solid line represents the unloaded hoof wall, the dashed line shows the change in shape that occurs during weight-bearing. Under load the dorsal wall flattens and moves palmarly, while the heels move laterally and caudally. GRF, ground reaction force. Reprinted from Douglas, J.E., Biddick, T.L., Thomasson, J.J. and Jofriet, J.C. (1998) Stress/strain behaviour of the equine laminar junction. J. Exp. Biol. 201, 2287–2297, with permission from the Journal of Experimental Biology, http://jeb.biologists.org/content/201/15/2287.full.pdf+html At impact the distance between the heels is wider than at rest, while at breakover, because of increased pressure in the toe area, the distance between the heels is narrower than at rest. Thus, the foot has three functional areas: the heels for damping; the wall, frog and sole for support; and the toe for propulsion (Barrey, 1990). Deformation of the foot during the stance phase optimizes these functions, and also enables the hoof to act as a pump for the blood circulation (Ratzlaff et al., 1985). The hoof mechanism has been studied using strain gauges (Knezevic, 1966; Bayer, 1973; Dyhre-Poulsen et al., 1994; Summerley et al., 1998; Thomason, 1998; Thomason et al., 2001, 2002; Roepstorff et al., 2001), photoelastic material (Davies, 1997; Dejardin et al., 1997, 1999), optical systems (Roepstorff et al., 2001; Burn & Brockington, 2006), doppler (Hoffman et al., 2001; Pietra et al., 2004), and special horse boots (Barrey, 1990; Preuschoft, 1989). As hoof strain and limb loading are related, the GRF can be estimated from hoof strain data using artificial neural networks in experiments where force plates cannot be used (Savelberg et al., 1997). Photoelastic materials did not show any concentrations of colored fringes in the foot of the standing horse following trimming (Davies, 1996). Shoeing stabilized (Thomason, 1998) or even decreased hoof movements (Colles, 1989b; Dyhre-Poulsen et al., 1994). Shoes with 5° raised heels reduce hoof deformation compared to flat horseshoes (Hinterhofer et al., 2000) whereas the extra frog pressure provided by heart bar shoes does not influence hoof expansion in a consistent manner (Colles, 1989a). The latter is in contrast to more recent work of Roepstorff et al. (2001) demonstrating that increased pressure on the frog and sole does increase heel expansion, although some heel expansion still occurs when the frog and sole are unsupported. Surprisingly, the digital cushion has no active role in this heel expansion (Taylor et al., 2005). Shoes with a toe clip and two side clips placed behind the 3rd nail (type of shoe used in the treatment of a fractured P3) minimize the hoof mechanism (Kersjes et al., 1985; Hinterhofer et al., 2001). Strains on the hoof wall are considerably higher at the trot than at the walk (Thomason, 1998) and are higher in the trailing limb than the leading limb at a gallop (Summerley et al., 1998). Surprisingly, riding decreases the strain in the quarters by 30%, while hoof wall strains are 20% higher on the medial side when the rider sits in the saddle and 20% higher on the lateral side for the forward seat (Summerley et al., 1998). Turning increases the hoof strain in the quarter that is on the inside of the turn by 40% (Summerley et al., 1998). A larger hoof angle and a longer toe length increased the strain at the toe, even when the length of the toe was proportional to the body size of the animals (Thomason, 1998). In the medial and lateral wall this relation was reversed: more upright feet were stiffer (Thomason, 1998). Elasticity of the hoof structures, however, is also affected by the moisture content: the wall consists of 82% keratin and 16% water, while the frog is 56% keratin and 42% water (Hertsch et al., 1996). Tubules are unlikely to be involved in the hydration status of the foot, but appear to have a more mechanical function in the hoof wall to redirect and resist cracks (Kasapi & Gosline, 1997, 1998). By tracking the path of the center of pressure in a craniocaudal plane it can be seen that immediately after initial ground contact it moves from the point of hoof contact to a position close to the apex of the frog (Barrey, 1990; Balch et al., 1997; Ovnicek, 1997; Van Heel et al., 2004). It remains there for most of the stance phase. At the end of the stance phase the center of pressure starts moving toward the toe. At toe-off it lies beneath the dorsal hoof wall at its breakover point (Barrey, 1990). Van Heel et al. (2005) studied the changes in location of center of pressure and hoof-unrollment pattern in relation to an 8-week shoeing interval and determined that horses can compensate to a certain extent for changes in hoof conformation that develop during 8 weeks on shoes. However, the capacity to compensate is less in the forelimbs and thus, the relative increase in loading of these limbs during a shoeing interval is larger compared to the hind limbs. The location of the center of pressure in a mediolateral plane shows that when the horse is standing squarely the direction is concentrated on the medial quarters (Colahan et al., 1991). During locomotion more force is recorded on the medial hoof with force shoes (Ratzlaff et al., 1993; Balch et al., 1997; Kai et al., 2000; Rollot et al., 2004; Roland et al., 2003; 2005, Chateau et al., 2009a), and with force and pressure plates in horses and ponies (Van Heel et al., 2004; Oosterlinck et al., 2010a,b,c). Transverse forces as recorded with force plates are not consistent throughout the gaits in the fore and hind limbs (Merkens & Schamhardt, 1994; Merkens et al., 1994; Weishaupt et al., 2002). Furthermore, extrasagittal joint motions in the forelimb will show bilateral variation since horizontal moments around the hoof center of pressure are not symmetric (Colborne et al., 2009). Changes in fetlock and coffin joint angulation affect strain in the palmar soft tissues that support the limb during the stance phase (Leach 1983; Bushe et al., 1987; Thompson et al., 1993; Riemersma et al., 1996a). The suspensory ligament (SL) and superficial digital flexor tendon (SDFT) are affected by the fetlock joint angle; strain increases as the fetlock extends. The distal accessory ligament (DAL) and deep digital flexer tendor (DDFT) are affected by the coffin joint angle: strain increases when this joint extends. The load distribution between the tendinous structures changes with alterations in hoof balance: strain reduction in one tendon may result in an increased strain in another structure. Also the horse may compensate, to a certain extent, for hoof imbalances by adjusting the length of the muscle bellies of the SDFT and DDFT or by changing the configuration of the joint angles in the proximal limb, e.g. when it experiences navicular bone pain in the distal limb (Wilson et al., 2001). Breakover is the terminal part of the stance phase from heel-off to toe-off. Rotation of the hoof is brought about as a result of tension in the DDFT and DAL, and in the navicular ligaments (Schamhardt et al., 1991; Riemersma et al., 1996b). Farriery modifications that facilitate breakover may reduce tension in the DAL and in the navicular ligaments and also reduce pressure of the DDFT against the navicular bone. The onset and duration of breakover are sensitive to changes in hoof balance, especially hoof angle and toe length. Hooves trimmed with a low heel and long toe have a significantly longer breakover duration, although other stride variables like stride duration do not change significantly (Clayton, 1988, 1990a,b). On the other hand, rocker, rolled and square toe shoes (Fig. 8.11) did not significantly alter breakover duration of horses trotting on a hard surface (Clayton et al., 1991; van Heel et al. 2006b) or on a rubber floor (Willemen et al., 1996). However, breakover tends to occur earlier (Eliashar et al., 2002) and is smoother with a lower peak loading (van Heel et al., 2006b; Fig 8.12). In contrast to the effect of heel wedges, shoes that facilitate breakover do not reduce the peak distal interphalangeal joint moment nor the absolute force exerted on the navicular bone (Eliashar et al., 2002). Off-center breakover has been associated with swing abnormalities of the foot that interfere with the opposite limb and contouring the hoof or shoe on one side are common techniques to correct for this. Recent research by Keegan et al. (2005) using gyroscopic sensors mounted on the hoof confirmed that shoes with a contoured lateral branch induce greater lateral roll during breakover. However, this effect was only observed at a trot and was limited to the first half of breakover. The flight arc of the hoof represents the summation of all the joint movements in the limb (Fig. 8.13). The highest point in the flight arc occurs soon after lift-off with a second, smaller elevation, which may coincide with an upward flip of the toe, at the time of maximal protraction. This gives a slightly biphasic flight arc (Clayton, 1990a; Balch et al., 1991a, 1997; Back et al., 1995). After the hoof leaves the ground, the limb swings forward to reach its position of maximal protraction, and is then retracted prior to contact with the ground. The final retraction is important for reducing the forward velocity of the hoof relative to the ground and so decreasing hoof deceleration at ground contact and preventing the horse from stumbling. Protraction of the limb is driven by muscles in the proximal limb, with the distal limb following passively (Back et al., 1995). In the forelimb the joints from the carpus proximally are driven by muscular action, while the fetlock, pastern and coffin joints move in response to inertial effects (Lanovaz et al., 1999). As maximal protraction is approached, the motion of the proximal limb is slowed and reversed by muscular action, while the distal limb continues moving forward until resisted by the passive structures (bones, ligaments, tendons). Swinging the limbs back and forth uses considerable energy, and a number of energy-saving mechanisms have evolved. One of the most important is the use of elastic structures as springs; energy is stored when elastic tissues are stretched as the limb is loaded during the stance phase, then released during unloading to bounce the limb off the ground and assist in flexing the joints. At the trot the SDFT, DDFT and SL are maximally stretched at midstance which corresponds with the time of maximal weight-bearing. Thus, the elbow, carpal and fetlock joints behave elastically during the stance phase at the trot (Clayton et al., 1998). Light-weight tactile stimulation devices attached around the pastern may increase the height of the flight arc and thus may represent a useful adjunct in physical therapy aiming at mobilization of joints and reinforcement of (atrophied) muscles (Clayton et al., 2011). However, short-term habituation does occur, especially in the forelimbs (Clayton et al., 2008). Application of the stimulators for short periods is recommended, although it is unknown how rapidly horses rehabituate to the effect of tactile stimulation of the pastern over different sessions. When a horse is trimmed with relatively long toes and/or short heels the hoof angle becomes more acute or sloping, while the pastern becomes more upright creating a broken-back hoof pastern axis (Bushe et al., 1987). Conversely, when horses are trimmed with a short toe and/or long heels, the hoof angle becomes more upright and the pastern angle becomes more sloping. Raising the heels decreases strain in the DDFT and DAL (Leach, 1983; Bushe et al., 1987; Thompson et al., 1993; Riemersma et al. 1996a), but increases strain in the SDFT and SL (Willemen et al., 1999b). One of the goals of hoof trimming is to achieve a flat landing with the objective of disseminating the forces on the foot as much as possible. When the hoof angle is more upright, the hoof has a more exaggerated heel first landing (Clayton, 1988; Back et al., 1995). Barrey (1990) looked at the relation between hoof angle and force distribution: 75% of the weight was borne by the heels when the hoof angle was 39°, and this was reduced to 57% when the hoof angle was increased to 55° (Fig. 8.14). This agrees with the finding that a larger toe angle results in more strain of the hoof wall at the toe (Thomason, 1998). Nevertheless, the quarters experience higher forces during stance than the toe (Barrey, 1990). Many racehorses are trimmed with an acute hoof angle because it is believed that the long toe low heel conformation enhances performance by increasing stride length. Comparison of the trot stride for a normal hoof angle versus an acute hoof angle showed no significant changes in stride length or suspension, and the flight arc of the hoof was almost identical with the two angulations (Clayton, 1990a; Balch et al., 1997; Girtler et al., 1995). However, the acute hoof angle was associated with an increased frequency of toe-first contacts, which was thought to be a consequence of the proprioceptive reflexes ensuring a fairly flat placement of P3 regardless of the shape of the hoof capsule (Clayton, 1990a). Since toe-first contacts are associated with a tendency to trip or stumble, this may be an undesirable effect. Decurnex et al. (2009) investigated the effect of training on proximal hoof circumference in young Thoroughbred racehorses being prepared for racing. Front hoof circumference immediately below the coronary band was measured weekly with a measuring tape in all horses present at the stable. Most horses showed a similar pattern of change. The proximal hoof circumference decreased during the training periods and increased when the horse was rested. Apparently, horses showed a decrease in circumference during race training that reversed when they were rested, thus contributing to a possible ‘environmental’ explanation for the long toe low heel conformation often found in racehorses. Furthermore, measurement of front hoof circumference is a simple method to assess change in hoof shape. It provides an opportunity to investigate the relationships between specific training, hoof shape and soundness (Peel et al., 2006).
The role of the hoof and shoeing
Introduction
Functional anatomy of the foot
General anatomy
Vascular supply
Nerve supply
Proprioception
Hoof mechanics in the standing horse
Hoof–pastern axis
Hoof balance
Craniocaudal balance
Mediolateral balance
Four point trimming
Hoof mechanics during locomotion
Initial ground contact
Impact
Hoof mechanism
Center of pressure
Breakover
Flight arc
Effects of hoof manipulations
Hoof angle
Stay updated, free articles. Join our Telegram channel
Full access? Get Clinical Tree
The role of the hoof and shoeing
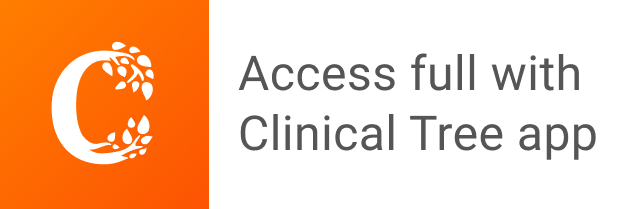