Chapter 13 Helen L. Birch, Pieter A.J. Brama, Elwyn C. Firth, Allen E. Goodship, José Luis L. Rivero and P. René van Weeren The horse has evolved from a forest-dwelling browser called Hyracotherium or Eohippus, which had four hoofed toes in the front feet and three in the hind feet and was not more than 8–9 inches (approximately 2 hands) at the withers, to the contemporary large ungulate measuring up to 18 hands. During the evolutionary process, which started in the Eocene and took some 60 million years, the horse changed from a typical forest inhabitant, which relied on hiding rather than flight for survival into an animal that was optimally adapted for living on the open steppe (Simpson, 1951). Through this process the locomotor system underwent profound change, as it became the most important factor in escaping from predators. The morphological changes that have imparted advantage for high-speed locomotion include the development of long and slender limbs with the muscles located proximally and close to the center of mass, reduced degrees of freedom of joint motion in the distal limbs, which grossly limits motion to the sagittal plane, and the use of collagenous components of muscle and tendon to reduce energy requirements in posture and locomotion. A very important athletic asset of the present-day horse is the storage of kinetic energy in the form of elastic energy in the tendinous structures of (especially) the limb flexors. This spring-like mechanism prevents energy loss and limits the role of the flexor muscles to dampening of these springs rather than using them to generate propulsion, thus greatly reducing the muscle volume needed (Wilson et al., 2001). The potential of the locomotor system led man to begin domesticating the horse in approximately 3000 BC (Dunlop & Williams, 1996). Unlike most other species, horses have always been used to increase the mobility and transport capacity of mankind, with more tangible products such as meat, milk and leather figuring as by-products only. Although the role of the horse in society has changed dramatically in the past 50–60 years in comparison to the previous five millennia, it is still its athletic capacity that gives the horse its role in present-day society. On a much shorter timescale, functional adaptation towards optimal locomotion capacity is also of crucial importance for the individual animal. The demands on the equine musculoskeletal system of many modern performance horses seem likely to be substantially more than those borne previously by animals principally used in transport, agriculture and the military, and are most probably much heavier than under most circumstances in the wild. The heavy challenges placed on the musculoskeletal system by present-day equestrian activities, together with the inevitable loss in robustness and sturdiness that goes with the process of domestication, may well lie at the heart of the high incidence of musculoskeletal injury and elevated wastage for orthopedic reasons in the equine species (Rossdale et al., 1985; Williams et al., 2001). Any musculoskeletal injury can be seen as a failure of the tissues to respond to the (mostly biomechanical) challenges placed upon them. Such failure may be the consequence of a high-impact single traumatic event, but more often follows the accumulation of repetitive micro-trauma. In the latter, training may be helpful to enhance the resistance of the musculoskeletal tissues to biomechanical loading. In fact, training is the artificial and purposeful enhancement of the degree of functional adaptation of tissues. Classically, there has been much interest in the effect of training on cardiovascular performance and on muscle strength, but other tissues respond to training too, though it should be clear that the extent of response of different tissues to the same training protocol may differ vastly. Another complicating factor is the age of the individual. Traditionally, research has been done in young horses, mostly aged 2–4 years, because of the early onset of the athletic career in the racing breeds and resulting commercial pressure. These horses cannot be classified as juvenile, nor have they yet reached full skeletal maturity (Gabel et al., 1977; Strand et al., 2007). There has been little interest in the effects of exercise on older horses, but some large studies have been conducted in the past decade on the effects of exercise in very young animals, e.g. in newborn foals. The latter research has made it clear that age indeed does matter. Whereas bone and muscle are known to respond to exercise throughout life, articular cartilage, tendons and ligaments are tissues that are much less, if at all, responsive in mature individuals. However, there is mounting evidence from recent research that these latter three tissues, which rank among the first with respect to injury prevalence and are notorious for their poor healing tendency, might be much more responsive to exercise in juvenile animals. This might open up new avenues for better adapting the equine musculoskeletal system to athletic challenge through programs of conditioning exercise at young age. However, such programs could be developed only if the exact effects of exercise on the diverse musculoskeletal tissues are known, if the exercise regimen to effect adaptation is known, and the appropriate time window in which to apply it. In the following chapter the major constituting tissues of the musculoskeletal system: bone, articular cartilage, tendons and muscle, are discussed with respect to their responsiveness to exercise. Discrimination is made between the effect of exercise in mature individuals and in the growing and still developing juvenile animal. Joint disorders have a major impact on equine athletic performance, as they rank either first or second as causes for wastage in performance horses, depending on the equestrian discipline involved (Rossdale et al., 1985; Todhunter & Lust, 1990; Williams et al., 2001). A joint is a complex entity that is made up of subchondral bone, articular cartilage, synovial membrane, and fibrous joint capsule and ligaments. Sometimes other structures such as a physis, tendon insertions, menisci or ligaments are present intra-articularly as well. Articular cartilage plays a pivotal role in the physiology of the joint because of its triple function: transmission of the forces generated by locomotion, acting as a shock absorber and providing a smooth gliding surface to enable supple joint motion. Articular cartilage has a crucial role in joint pathology, as in most joint disorders it is the principally affected joint tissue and has, at least in mature individuals, a very limited capacity for repair. In the following section first a brief synopsis will be given of some essential principles of articular cartilage biology, followed by a paragraph on the developmental aspects of articular cartilage, which are essential to appreciate the effects of exercise on young, growing individuals and the possible implications thereof. The section ends with a paragraph on the effects of exercise (or lack thereof) in more mature individuals. Articular cartilage plays an important role in both the transmission and attenuation of forces generated by locomotion, which leads to seemingly contradicting requirements of both elasticity and great strength. Nature provides a solution to this problem by conceiving an extracellular matrix (ECM) that consists of two principal components: collagen fibrils and proteoglycan aggregates. The collagen fibrils are made up of collagen type II molecules (3 α1-chains in triple helix configuration) and form a three-dimensional arcade network, as was first described by Benninghoff (1925). The fibrils start from the layer of calcified cartilage just above the subchondral bone, then course perpendicular to this layer towards the joint surface and bend back, running tangentially to the joint surface and directly under it, to close the arch by returning perpendicularly again to the calcified cartilage layer (Fig. 13.1). Cross-links that interconnect α1-chains within the same collagen molecule and interconnect collagen molecules and different collagen fibrils provide structural coherence and extra strength. Cross-link formation is one of the so-called post-translational modifications of collagen and is the last chemical modification that occurs during the formation of the primary collagen structure. There are various types of cross-links with the pyridinoline cross-links that form between lysyl and hydroxylysyl residues in the collagen network (lysylpyridinoline or LP-cross-links and hydroxylysylpyridinoline or HP-cross-links respectively) as the most common covalent cross-links. Cross-links have a major influence on structural and consequently biomechanical characteristics of the collagen network (Eyre & Wu, 1995) and the formation of cross-links is mainly an irreversible process (Ray et al., 1996). A special category of cross-links is formed through the process of non-enzymatic glycation. As collagen molecules have an exceptionally long lifetime once incorporated into the ECM of cartilage (see later), they are susceptible to the accumulation of advanced non-enzymatic glycation end products (AGEs) via the so-called Maillard reaction (Monnier, 1989). The process results in increased cross-linking, such as pentosidine formation from lysine, sugar and arginine moieties. Pentosidine is one of the few Maillard cross-links of which the structure has been elucidated, and is used as a sensitive marker for the entire process (Vlassara et al., 1995). The accumulation of AGEs depends on the turnover rate of a protein or tissue and is thus a measure for the metabolic rate of that structure (Verzijl et al., 2000). Fig 13.1 Schematic drawing of articular cartilage showing the arcade-like architecture of the collagen fibrils as first described by Benninghoff (1925). Typically, the collagen fibrils emerge from the calcified layer perpendicular to the tidemark and start to arch in the intermediate zone, to become aligned with the articular surface in the superficial zone. Interspersed between the collagen fibrils and connected to them either directly or via hyaluronan or hyaluronic acid molecules are proteoglycan aggregates, of which aggrecan is the most important. Aggrecan consists of a core protein with large numbers of sulphated glycosaminoglycans (chondroitin sulphate and keratan sulphate) as side chains. Because of their sulphate groups, these side chains are negatively charged and attract water. The combination of the swelling pressure of the sulphated glycosaminoglycans and the confinement due to the stiffness of the collagen network creates an intrinsic pressure within the ECM of articular cartilage that gives it the unique combination of resilience under compression and great resistance against tensional and shear forces (Todhunter, 1996). Different areas of articular cartilage are subject to different types of loading, such as low-level constant loading, intermittent loading, and very high impact loading (Fig. 13.2). These variable loading conditions can only adequately be met, without being damaged in the long term, by cartilage possessing different mechanical properties (Herzog & Federico, 2006; Palmer & Bertone, 1996) through appropriately different biochemical and ultrastructural characteristics over the cartilage surface (Brama et al., 2000b,c; Brommer et al., 2005; Murray et al., 2001a; Palmer et al., 1995a,b) (Fig. 13.3). Two sites of the proximal first phalangeal bone, known to be subjected to strongly differing loading conditions and exhibiting a difference in predisposition to cartilage disease, have received considerable attention with respect to topographical heterogeneity in cartilage composition and loading characteristics (Brama et al., 1999b, 2001, 2002) (Fig. 13.4). Cartilage also displays zonal heterogeneity along its depth. The superficial layer contains flattened chondrocytes, has densely packed type II collagen fibrils oriented parallel to the surface, a small amount of proteoglycans (PG) and high water content (Aydelotte et al., 1988; Aydelotte & Kuettner, 1988). The middle (transitional) zone has lower water content, a higher concentration of PG, and a lower concentration of collagen fibrils that are less organized, and rounded chondrocytes dispersed irregularly in the extracellular matrix. The deep zone has collagen fibrils oriented perpendicular to the articular surface, the highest concentration of PG, lowest percentage of water of any zone, and chondrocytes arranged in columns perpendicular to the subchondral bone (Todhunter, 1996). Articular cartilage is, like any living tissue, not a static entity but undergoes constant remodeling through anabolic and catabolic processes. The chondrocyte, the only cell type found in normal articular cartilage, produces all ECM components. Collagen molecules are produced intracellularly as pro-collagen and are extracellularly modified by enzymatic cleavage of the C- and N-terminal propeptides before they are integrated in the collagen network. Degradation of ECM is effectuated by various proteinases, of which the matrix metalloproteinases (MMPs) and aggrecanases (members of the disintegrin and metalloproteinase with thrombospondin motifs (ADAMTS) gene family) are the most important. However, the principal constituents of the cartilage ECM, collagens and proteoglycans, have very different turnover rates. Normal turnover time of proteoglycans is variable and ranges in mature individuals from about 300 days for overall turnover in canine articular cartilage to around 20 years for the long-lived globular hyaluronic acid binding domain of aggrecan in human cartilage (Maroudas, 1980; Maroudas et al., 1998; Verzijl et al., 2001). However, collagen turnover times have been estimated to be up to 350 years in mature human cartilage (Maroudas et al., 1992). Comparable data for the horse do not exist, but are assumed to be in the same order of magnitude. It is this very low turnover rate and thus extremely limited repair capacity that lies at the basis of the famous observation by William Hunter (1743) that ‘ulcerated cartilage when destroyed, will never repair’, and that makes OA in both humans and horses into such a debilitating disease with a very poor long-term prognosis. Theoretically, there are two possible origins of topographical heterogeneity of articular cartilage biochemistry: genetic predetermination or formation during early life when cartilage metabolism is still high during active growth. The first indication that the latter could be true came from a study, which showed that proteoglycan content was identical all over the joint in neonatal lambs, unlike the situation in adult sheep where there is topographical heterogeneity (Little & Ghosh, 1997). The same was true for the horse, in which proteoglycan content and collagen, including post-translational modifications of collagen such as cross-links, were homogeneously distributed, at least when looking at average values of full thickness samples, across the joint of the newborn in what has been called a ‘blank joint’ (Brama et al., 2002). Most of the topographical heterogeneity appeared before the age of 5 months, but HP cross-links (the most abundant pyridinoline cross-link in articular cartilage) did not reach their mature values until after 12 months (Brama et al., 1999a, 2000b, 2002). Recently, this maturation process of articular cartilage in early life was confirmed by an investigation using equine-specific cDNA micro-arrays. The expression of genes encoding matrix proteins and matrix modifying enzymes was clearly different between neonatal and mature individuals with the former expressing more collagens, matrix-modifying enzymes and provisional matrix non-collagenous proteins and the latter showing a transition from growth to homeostasis and tissue function related to coping with shear and compressive forces (Mienaltowski et al., 2008). The changes in biochemical composition of the extracellular matrix of articular cartilage during early life are accompanied by structural changes. The arcade structure of the collagen network as described by Benninghoff (1925) is typical for mature cartilage, but not yet present in the newborn. Polarized light microscopy has shown that at birth collagen fibril orientation is predominantly parallel to the joint surface and subchondral bone and then gradually changes to the more classic Benninghoff arcade structure (Rieppo et al., 2008). Preliminary data from the horse suggest that here too the mature configuration is basically formed during the first 5–6 months of life (van Turnhout et al., 2008). If the topographical heterogeneity of the extracellular matrix of articular cartilage enables the joint to meet the biomechanical challenges to which it is subjected and if this topographical heterogeneity is formed from scratch in the young individual in the so-called process of functional adaptation (Brama et al., 2002), then biomechanical loading is the most likely driving force of this adaptive process. The first evidence that this is true came from a study that was originally designed to study the influence of exercise on the developmental orthopaedic disease osteochondrosis (van Weeren & Barneveld, 1999a). In that study, named EXOC, a group of 43 Warmblood foals was divided into three groups from the age of 1 week. One group (boxed; n = 14) was kept in box stalls for 24 h/day; the second group (boxed/sprinted; n = 14) was kept in similar box stalls, but given exercise in the form of a number of short sprints during a restricted period, i.e. in this group high-intensity exercise was alternated with a very sedentary lifestyle; the third group (pastured; n = 15) had free pasture exercise for 24 h/day. The exercise regimens were maintained until weaning at 5 months of age, at which age 8 foals from each group were euthanized and their tissues harvested for biochemical and other analyses. The remaining 19 foals were then kept together in a single group and allowed a moderate exercise regimen. These animals were euthanized at age 11 months and had their tissues analyzed to see if any difference provoked by the different exercise regimens at 5 months would remain or was reversible once a similar exercise regimen was given to all foals (Fig. 13.5). The study showed that topographical heterogeneity, expressed as a significant difference from zero of the ratio of two very differently loaded sites within the joint, developed in the pastured group and in the boxed/sprinted group alike, but failed to develop in the boxed foals. The most interesting observation, however, was that, where proteoglycans became normal after the common training program from 5 to 11 months (as did exercise-induced differences in other tissues from the same experimental animals such as bone mineral density (Cornelissen et al., 1999; Firth et al., 1999b)), levels of collagen or the collagen-related hydroxylysine levels remained abnormal and failed to develop the topographical heterogeneity that is characteristic of mature individuals (Fig. 13.6) (Brama et al., 2002). Therefore, whereas this study unequivocally demonstrated the importance of biomechanical loading for the development of the topographical heterogeneity of the extracellular matrix, it showed also that, at least with respect to the collagen component, there is a limited window in time when this process of functional adaptation can take place. This stresses the importance of sufficient exercise in the early juvenile phase. Fig 13.6 The influence of different exercise levels during the first 5 months after birth on the collagen ratio of site 1/site 2 (see Fig. 13.4). Values are given as mean ± standard error of the mean. Pasture: pasture exercise; training: box rested/sprinted animals; box rest: box rested animals (see also Fig. 13.5). n, number of animals; *, p < 0.01. Note that both groups that were exercised develop a site 1:site 2 ratio that is significantly different from one in the first 5 months of life, indicates that they developed topographical heterogeneity. The box rested animals do not develop this topographical heterogeneity and fail to compensate for this in the following 6 months when undergoing a moderate exercise regimen. Reproduced from Brama et al., 2002. Equine Veterinary Journal 34, 265–269., with permission. Another interesting observation was made in the boxed/sprinted animals that had been subjected to a combination of short bouts of high-intensity exercise with a sedentary lifestyle. The degree of development of topographical heterogeneity in this group was comparable to the pastured animals, which were regarded as controls. However, when culture experiments were carried out with chondrocytes harvested at age 11 months from animals from the former exercise groups, it appeared that chondrocytes from the former box/sprinted group could not be stimulated to increase metabolic activity, in contrast to samples from both other groups. This was interpreted as a deleterious long-term effect of the combination of sedentarism with bouts of high intensity exercise, possibly representing a kind of cellular exhaustion that might be due to overstimulation during the first 5 months of life (van den Hoogen et al., 1999). Similar phenomena were observed in other tissues from these animals (Barneveld & van Weeren, 1999). Also, forced exercise (box/sprinted) had a negative effect on collagen turnover, as measured by serum markers CPII and Ctx1, when compared to pastured foals (Billinghurst et al., 2003). Another large-scale exercise study concerning foals was conducted by the Global Equine Research Alliance (GERA, a consortium of the equine orthopedic research groups from Massey University, New Zealand, Colorado State University, USA, Royal Veterinary College, UK and Utrecht University, the Netherlands). This study became known under the acronym GEXA. In the GEXA study, 33 Thoroughbred foals were raised from 0–18 months under different exercise conditions. One group (PASTEX, n = 15) had free pasture exercise all year round, in agreement with common New Zealand practice; the second group (CONDEX, n = 18) was raised under similar conditions, but subjected to an additional exercise program as well. The exercise program consisted of cantering and sprinting on a track which increased overall workload by a moderate, but significant, 30% (Rogers et al., 2008a). At age 18 months, 12 animals (six from each group) were sacrificed and their tissues harvested for detailed analyses. The remaining animals were broken in and trained and raced as 2- and 3-year-olds (Rogers et al., 2008b) (Fig. 13.7). Fig 13.7 Schematic diagram of the experimental set-up of the so-called GEXA study. Thirty-three Thoroughbred foals were divided into 2 different exercise groups from average age 3 weeks until age 18 months. Exercise regimens consisted of 24 h/day pasture exercise (PASTEX, n = 15), or pasture exercise with additional track training that increased workload by 30% (CONDEX, n = 18; for details of training protocol see Rogers et al. 2008a). At age 18 months, six animals from each group were euthanized and their tissues harvested. One PASTEX animal was lost due to an accident and the remaining 20 animals were trained for racing as 2- and 3-year-olds. Cross symbol indicates euthanasia. The articular cartilage of the metacarpophalangeal and metatarsophalangeal joints from the GEXA animals harvested at 18 months was meticulously researched using a variety of techniques. Biochemical and biomechanical analyses of full-thickness samples from the distal metacarpal bone showed site-related differences, but no exercise effect (Nugent et al., 2004). The same applied to the metabolic rate of chondrocytes (measured by 35S-uptake) from third metacarpal cartilage (Kawcak et al., 2010). However, the exercised horses had more viable chondrocytes in the more heavily loaded sites than in the less loaded sites of the same metacarpal cartilage (Dykgraaf et al., 2008). Also, a detailed study of contiguous approximately 100-µm thick slices taken from the proximal articular surface of the proximal phalanx down to the tidemark at differently loaded sites (see above) showed not only obvious site differences, but also exercise-related changes. There was no exercise effect on proteoglycan content, indicating that the exercise level had not been strenuous and confirming the work by Nugent et al. (2004) and Kawcak et al. (2010); but at 18 months old the normal physiological increase in collagen at the site located at the joint margin was less in CONDEX animals (Brama et al., 2009a). This was interpreted as a precocious cessation of collagen remodeling at this site due to advancement in time of the normal maturation process. The biochemical analysis of similar slices from the same site in the metatarsophalangeal joint pointed in the same direction: in the CONDEX animals, hydroxylysine, HP cross-links and pentosidine cross-links were all higher, all indicative of advancement of the normal process of functional adaptation (van Weeren et al., 2008). The increased pentosidine levels in the CONDEX animals are of particular interest, because they indicate a lower metabolic activity in this group, confirming more rapid progression of the physiological age-related decrease in matrix turnover and thus indicative of an advanced degree of maturation compared to the PASTEX animals (Fig. 13.8). Other evidence for the difference in maturation rate came from ultrastructural and biomechanical studies. Polarized light microscopy techniques were used to investigate the spatial arrangement of the fibrils of the collagen network throughout the depth of the cartilage, measured as parallelism index (PI, a measure of the degree to which the collagen fibrils are aligned with each other) and orientation index (OI, a measure of the average angle of collagen fibrils with respect to the articular surface). Parallelism index was higher in CONDEX animals, again indicating advanced maturation (Brama et al., 2009b) (Fig. 13.9). An interesting observation was that orientation of collagen fibrils in the deep zone of the cartilage was less perpendicular than expected, which is possibly an adaptation of the direction of collagen fibers to high shear forces in the equine metacarpophalangeal joint, which is a heavily loaded joint with an exceptionally high range of motion where an oblique insertion of the collagen fibers in the calcified cartilage layer may yield better resistance to repeated shear forces than would a perpendicular configuration. Based on this observation it was hypothesized that the Benninghoff arcade model may be more flexible than commonly thought and that local adaptation includes a predominantly non-perpendicular direction of the collagen fibrils in the deep zone. Biomechanical analysis of samples from different sites showed that in CONDEX animals site-related differences known to develop with age (Brommer et al., 2005) were less marked than in PASTEX animals with respect to Young’s modulus (ratio of axial stress and strain in unconfined compression) and dynamic modulus (proportional to the elastic and viscous energy dissipated in the loading process and essentially a measure of dynamic stress and strain (Palmer & Bertone, 1996)). This may have been caused by earlier down regulation of collagen metabolism in trained animals, as signaled earlier (van Weeren et al., unpublished results). The quicker maturation of the collagen matrix and associated other pattern of topographical heterogeneity need not necessarily be deleterious, as the same study showed that the cartilage degeneration index (CDI) as described by Brommer et al. (2003) was significantly less in the CONDEX animals, indicating better surface integrity in this group (van Weeren et al., unpublished results). There is also evidence from other species that the exercise regimen at early age may affect cartilage properties and that this effect need not always be reversible. Kiviranta et al. (1987) showed in young Beagles in which one of the hind limbs was immobilized for 11 weeks that GAG content may be reduced by almost 50% in the non-weight-bearing limb, whereas cartilage thickness and GAG-content was increased in the contralateral limb. When the study was repeated, but now followed by a 15-week rehabilitation program, the normal situation was not fully restored; the authors concluded that immobilization of skeletally immature joints may affect cartilage development such that recovery is very slow or alterations are permanent (Kiviranta et al., 1994). No large studies have been conducted on the effect of exercise on cartilage from completely mature or elderly horses, but work has been performed on 2-year-old Thoroughbreds. These animals can be classified as ‘young adult’, although they cannot be considered as fully skeletally mature yet because they are still growing (Green, 1976), although at a much lower rate than the foals that were discussed in the preceding paragraph. In the so-called short-term Bristol study 2-year-old Thoroughbreds were subjected to a 19-week exercise regimen that consisted of three times/week galloping on a treadmill, trotting in a mechanical horse walker three times weekly and 40 min walking exercise six times weekly. The control group was only walked for 40 min six times per week during the same period (Murray et al., 1999b). In the MUGES study (Massey University Grass Exercise Study), a group of 2-year-old Thoroughbreds was trained for 13 weeks on grass and sand tracks, after which their musculoskeletal tissues were analyzed (Firth et al., 2004a). Cartilage taken from the proximal articular surface of the radial, intermediate and 3rd carpal bone from exercised animals that had participated in the Bristol study had a thicker calcified, but not hyaline cartilage layer than from non-exercised animals (Murray et al., 1999a). The cartilage of the strenuously trained animals was biomechanically less stiff, showed more fibrillation and more chondrocyte clusters than the gentler trained animals, suggesting that strenuous exercise may lead to deterioration of cartilage (Murray et al., 1999b). Also fibronectin (Murray et al., 2000) and cartilage oligomeric matrix protein (COMP) distribution (Murray et al., 2001b) appeared to be influenced by exercise. When cartilage from animals of the 19-week Bristol study was cultured to measure chondrocyte metabolic activity, proteoglycan synthesis rates were higher in the trained animals than in the controls, confirming the overall effect of strenuous exercise on the proteoglycan component of articular cartilage ECM (Bird et al., 2000). There were no overall effects on collagen levels, but sites predisposed to clinical signs contained significantly less collagen in horses undergoing the exercise program; also, strenuously exercised animals had higher glycosaminoglycan content than horses from the more gently trained group, which was most marked in the cartilage from the dorsal radial and dorsal intermediate carpal surfaces, sites known to be heavily loaded during exercise (Murray et al., 2001a). Mechanistically, the hypothesis that the degrading enzymes Cathepsin B and D might be involved in the exercise-induced alterations could not be confirmed, but it was demonstrated that the two enzymes were regulated differently by mechanical loading (Bowe et al. 2007). In contrast to the Bristol study, the MUGES study found a significant increase in thickness of (hyaline) cartilage after 13 weeks of training (Firth & Rogers, 2005), compared to pasture-raised controls, in particular in sites within the intercarpal joint. At these sites there was no patho-anatomical damage, leading to the conclusion that the response of the tissue was adaptive and not degenerative in nature. However, there was a difference between joints, because in the metacarpophalangeal (MCP) joints of the same animals wear lines and fibrillation were present and water content was increased while HP cross-links had fallen compared to the controls, which was interpreted as signs of loosening of the collagen network due to micro-damage (Brama et al., 2000a). The metacarpophalangeal joint is known to be the equine joint most susceptible to damage (Pool, 1996) and predilection for patho-anatomical damage in the MCP joint has been shown in both treadmill-exercised horses (Kawcak et al., 2000) and in wild horses (Cantley et al., 1999). Also the study by Little et al. (1997) showed that there is a level at which exercise may become deleterious. In an ex vivo study in which material from the dorsal radial facet of the third carpal bone, an area well-known to be subjected to high contact stresses in galloping horses, was cultured that came from horses having undergone a strenuous exercise regimen, a significant reduction in aggrecan synthesis and a concomitant increase in decorin synthesis could be determined compared to less vigorously trained controls. The suggestion was made that this alteration in articular cartilage metabolism could be a predisposing factor for cartilage degeneration and OA at a later stage. An effect of exercise on the biomechanical properties of cartilage was shown in a relatively short (6 weeks) treadmill study (Palmer et al., 1995a), in which the permeability constant increased at all sites investigated, but the Poisson’s ratio increased only at specific sites, stressing the topographical variation in response to biomechanical stimuli, which is most probably related to local differences in joint architecture and geometry. The overall metabolism-enhancing effect of exercise on articular cartilage has been studied indirectly in several studies that focused on a variety of potential biomarkers in either serum or synovial fluid. Plasma GAG levels, and serum keratan sulphate and COMP all increased after moderate to strenuous exercise (Calatroni et al., 2008; Okumura et al., 2002; Helal et al., 2007). Brown et al. (2007) looked in more detail into changes in glycosaminoglycan metabolism due to exercise and found higher chondroitin sulphate peak chain lengths, but shorter hyaluronic acid chain lengths in exercised versus rested horses. Investigating both serum and synovial fluid, Frisbie et al. (2008) confirmed the increase in proteoglycan markers after exercise, and showed that the same was true for a number of collagen markers. However, moderate exercise did not affect MMP-1 activity in synovial fluid (Brama et al., 2004). There is a reciprocal interaction between synovial fluid and articular cartilage and the former is not just a reflection of the status of the latter. The effects of joint loading on cartilage are, at least in part, mediated by alterations in the synovial fluid, as cartilage explants cultured in post-exercise synovial fluid showed enhanced GAG synthesis and diminished release when compared to cultures using pre-exercise synovial fluid (van den Hoogen et al., 1998). Also in other species there is evidence for an influence of exercise on articular cartilage, at least in young individuals. In young Beagle dogs, moderate loading levels tended to increase GAG production, while strenuous exercise led to GAG depletion in high-load areas (Arokoski et al., 1993; Kiviranta et al., 1988, 1992, 1994; Säämämen et al., 1994). In man it has been shown that children not undergoing programs of vigorous activity had approximately 25% less cartilage than children that had been mildly active (Jones et al., 2003). There is no doubt that articular cartilage is responsive to biomechanical loading and that responsiveness is highest in the youngest animals. In fact, there is now substantial evidence that the characteristic topographical heterogeneity in composition and ultrastructure of the extracellular matrix of mature cartilage is formed under the influence of loading, i.e. through physical exercise. It has been suggested, therefore, that the collagen network of juvenile animals that are in a phase of rapid growth might respond to loading in a fashion similar to that in which trabecular bone is known to respond according to Wolff’s law (van Weeren & Brama, 2003). The difference with bone is that, whereas bone will continue to respond to exercise throughout life, the collagen network can most probably do so only in the young, growing animal before the collagen remodeling rate falls to the extremely low level related to the very long collagen turnover times in mature animals mentioned earlier. Indeed, although some studies have shown a response of the proteoglycan component of the ECM of cartilage to exercise in (young) adult horses, no adaptive response of collagen has ever been demonstrated in this age group. This makes the early juvenile period the only window of opportunity to manipulate the collagen network through modification of loading, e.g. differences in exercise. Recent studies show that such manipulation is possible and may irreversibly delay the normal maturation process as the result of a decreased exercise level or lead to accelerated maturation resulting in a different topographical pattern in animals subjected to more exercise than free pasture exercise. There is thus reason to believe that exercise at an early age may indeed have long-lasting effects on the collagen matrix of articular cartilage, lending credibility to the hypothesis that variations in exercise regimens of juvenile persons or animals may influence the risk for the development of degenerative joint diseases later in life (Helminen et al., 2000). This may mean for the horse that it might be better to start athletic training rather than later, as has been suggested (Smith et al., 1999). However, long-term epidemiological studies are necessary to establish whether differences in the development of the biochemistry and ultrastructure of articular cartilage that are now known to be modulated by early exercise have significant implications for the orthopedic health and/or performance of the equine athlete during its career. Bone is the tissue that confers rigidity to the musculoskeletal system and its intimate association with articular cartilage allows both tissues to sustain the large forces generated by normal and athletic locomotor activity. Bone derives its resistance to deformation from the hydroxyapatite crystals that are laid down on a collagen matrix, providing the tissue with great strength, but also reducing elasticity substantially. The responsiveness of bone to biomechanical influences has been recognized universally since the late 19th century when Julius Wolff proposed his law, stating that bone will adapt both architecturally and with respect to composition to changes in mechanical loading (Wolff, 1892). Failure of bone results in (micro)fractures. The incidence of bone failure in equine orthopedics varies strongly with the discipline involved, but can be substantial, particularly in racing (Rossdale et al., 1985; Williams et al., 2001). Clinically, bone failure in the horse may vary from relatively mild, yet economically important, micro-fractures and fissures as seen in sore shins (Boston & Nunamaker, 2000), to catastrophic fractures of long bones (Parkin et al., 2006). In the following section an introduction on the morphology and physiology of bone is given first, followed by a discussion of the influences of exercise on bone in general and more specifically for the horse. Most of the earlier work has been done in young adult animals; recently the effect of exercise on foals has become an area of interest. The diaphysis of long bones has a marrow cavity, which allows a better strength/weight ratio to resist bending and torsional forces, while positively affecting gait velocity by minimizing the weight of the distal limb. Most features of a long bone and cuboidal bone are illustrated in Figure 13.10. There is a gradual transition from the dense bone of the diaphyseal cortex to the metaphysis, in which the junction of the outer compact bone with the cancellous bone is indistinct. The epiphysis is contained within a dense shell of compact bone, which in articulating regions is referred to as the subchondral bone (SCB), is regionally thickened at various sites, and has a gradual transition into the bulk epiphyseal trabecular bone (BETB). The orientation and architecture of the trabecular bone of the metaphysis and epiphysis is central to how load is transferred from the diaphyseal cortex to the joint surface and thence across the joint. The metaphyseal cortex is thin, and this is most obvious close to the physis, and especially in the young presumably because of the rapid growth rates in early life. The cross section of the whole bone is greatest at the level of the physis, and the contact area of the articulating surface of the bone with its opposing counterpart in the close-packed position is also large. This maximization of the force-bearing surfaces allows articular and physeal cartilage and immature bone to sustain lower stress, although the force transmitted across the joint and through the physis and metaphysis equals that borne by the diaphysis, which is much more dense and much smaller in cross-section than elsewhere in the bone. The extracellular matrix of bone is made up of a framework of osteoid, consisting of about 90% collagen (type I), around and in which hydroxyapatite crystals are precipitated. After suitable stimulation, the osteoid is 70% calcified within a period of days, but complete mineralization takes several months (Hayes, 1991). About 50% by volume and 75% by weight of bone is mineral, consisting mostly of calcium phosphate and calcium carbonate in the form of hydroxyapatite. The importance of collagen in determining bone strength has been rarely included in analyses of variance of bone strength, most likely because bone mineral density parameters became dominant, being so easy to measure. Conversely, quantification of collagen in bone was, until recently, much more difficult and required invasive sampling. But the central importance of collagen and its orientation is clear since bone mineral is deposited in and on collagen; and the directionality of the collagen fibrils determines the orientation of the mineral plates or spindles (Wassen et al., 2000; Viguet-Carrin et al., 2006). Compact cortical bone contains spaces within it, including osteocyte lacunae, blood vessels in Haversian and Volkmann canals, and resorption spaces, the volume of which varies with age, recent loading regimen, metabolic status, injury, endocrine status, and lactation. Bone matrix is the same material in diaphyseal cortex and a single trabecular plate. However cancellous bone tissue has a bone volume fraction (proportion of total volume occupied by bone) of 0.47–0.82 in the normal third carpal bone (Young et al., 1991a), which is significantly less than the range 0.92–0.98 for the mid-radius cortex (Riggs et al., 1993), because the inter-trabecular spaces are far larger than the spaces in the cortex. Within (any given) whole bone, the amount of mineral will differ between its regions, due to differences in the relative amount and placement of cancellous and cortical tissue at different sites. The word ‘bone’ is applied to three different entities: bone material, bone tissue consisting of bone material plus the spaces and tissues interposed around the material, and bone as an ‘organ’ or ‘whole’ bone. The three entities have different mineral densities, which have been referred to as material, compartment, and total bone mineral densities respectively (Rauch & Schoenau, 2001). Most methods used to assess the response of bone to exercise are based on quantifying the amount and disposition of mineral within the bone. The mechanical properties of bone are closely related to mineral in the matrix, and the modulus of elasticity is directly related to volumetric bone mineral density (BMDv) of the bone tissue (Currey 1988b; Ferretti et al 1996), at least within the physiological range of BMDv. Collagen orientation and cross-linking also affect variance in mechanical properties of bone (Riggs, 1993; Oxlund, 1995; Viguet-Carrin et al., 2006), but the extent to which collagen parameters affect bone strength, and when this is rate limiting in the growing and training horse if injury is to be prevented, have not been established. Bone responds to exercise in one or more possible ways. These include increase in mineralized mass (Goodship et al., 1979; Woo et al., 1981; Gordon et al., 1989), increase in bone material density (Boyde & Jones, 1996), increase in bone mineral density of the whole bone (Cornelissen et al., 1999; Firth et al., 1999b, 2000), change in trabecular bone density and orientation (Young et al., 1991b; Firth et al., 1999a,c) alteration in remodeling, and increase in maturation of secondary osteons (Reid & Boyde, 1987), change in geometry through size increase as a result of changed endosteal and subperiosteal apposition rates (Gordon et al., 1989; Kontulainen et al., 2003), change in collagen cross-link concentrations (Oxlund et al., 1995; Köwitz et al., 1997), change in predominant collagen fiber orientation (Martin & Ishida, 1989; Boyde & Riggs, 1990; Martin & Boardman, 1993; Riggs et al., 1993) and change in bone collagen synthesis and remodeling (Tidswell et al., 2008). The diaphysis of immature individuals consists of primary osteonal bone embedded between more highly mineralized concentric plates of woven bone. Bone formed post-natally at the periphery is more porous, contains wider, less mineralized lamellae, and has osteons of greater diameter than does cortical bone formed pre-natally. The bone shaft can respond to exercise by enlargement as a result of the formation of woven-fibred bone in the periosteum, which then forms lamellar bone and becomes connected by radially oriented bony plates, with apposition of bone leading to new primary osteons (Stover et al., 1992). This allows prompt and rapid periosteal new bone formation, with an increase in resistance of the bone to bending and torsion. Compared with this periosteal response in the diaphysis and metaphysis, the capability for epiphyseal enlargement in response to the biomechanical stimuli is apparently limited, in both extent and rate, and occurs largely in early life. The development and maintenance of tissues requires the delivery of a certain number of cycles per day at certain strain rates, in order to stimulate various tissues: for instance, in young rats taught to jump, five cycles per day of this unusual activity produced as great a response in terms of resistance to fracture than did 40 jumps (Umemura et al., 1997). The effect on other tissues and species may differ, and each may have its own stimulus requirements if it is to adapt. Unfortunately, the number of other activities that deliver these novel higher forces to tissues is not documented in either domesticated or wild horses. It is assumed to include the playing, fighting, fast locomotion and other exuberant activities that young mammals engage in habitually and adults less often. The duration of these high-intensity exercises need not be long. The natural grazing and social slow movement behavior of wild horses is known to occupy ~17 h/day, whereas Dutch Warmblood foals at pasture did not canter more than approximately 3 min per day (Kurvers et al., 2006). This lack of knowledge is one reason for the current interest in the area of exercise in the young foal. But there is also little research in the area of training for particular athletic objectives. There is close similarity of equine structure and function to that in people, and there is increasing interest from the human health community in the responses of the tissues of children to exercise, or the lack of it. The outcome parameters that have been used to quantify a bone response include radiography, radiogrammetry, radiographic bone aluminium equivalence, point projection radiography, dual X-ray absorptiometry (DXA), computed tomography (CT), peripheral quantitative computed tomography (pQCT), magnetic resonance imaging (MRI) (Firth, 2004), and clinical and pathological features associated with, for instance, cartilage and subchondral bone lesions related to imposition of exercise. The latter are not considered in this chapter. Several aspects of bone development became evident in an investigation (EXOC study) of the relationship between exercise and osteochondrosis in Warmblood foals. Three groups of foals were kept from birth for 5 months in one of three exercise conditions: in a box stall with the mare (boxed group), boxed but exposed to sprint exercise 5 days per week in a 50-m long walled enclosure from a young age (boxed/sprinted), or kept at pasture continually (pastured). After euthanasia of some foals at 5 months of age, the remaining foals until 11 months of age were out of the weather in a large stall, which was always open to a small enclosure. The change in exercise regimen meant that the previously boxed group had greater opportunity for exercise, the previously boxed/sprinted and pastured groups had less (van Weeren & Barneveld, 1999a). At 5 months, pQCT showed that the cross sectional area (CSA) of the third metacarpal bone (Mc3) was significantly less in the boxed than pastured group, but the BMDv was not different between groups, although BMDv in the dorsal cortex was significantly higher in the boxed group, possibly due to a difference in the remodeling rate. The between-group differences had disappeared in the foals aged 11 months, and the pooled values at 11 months for area were higher and for density were lower than at 5 months (Cornelissen et al., 1999), although there was no decrease in other foals pastured from birth, and scanned at 6 and 9 months of age (Grace et al., 2003), possibly due to different experimental design and technique (Firth, 2006). In the proximal sesamoid bone, the trabecular BMDv was highest in the boxed/sprinted group, and lowest in the boxed group, and the value in the latter increased greatly after the exercise regimen changed at 6 months if age. The BMDv of the previously pastured group had increased by 11 months, but that of the previously boxed/sprinted group had not. Confinement apparently had resulted in a retardation of normal development which was compensated for, at least partly, when the restriction on exercise was lifted (Cornelissen et al., 1999). The BMDv of the medial aspect of the third carpal bone was significantly less in boxed foals at 5 months old, with the effect of sprint training being similar to that of pasture exercise. By 11 months old, the reduced exercise possibility in previously pastured and boxed/sprinted groups and the greater exercise in previously boxed foals were associated with a significantly greater BMDv than at 5 months old, but no difference between groups. The average dorso-palmar depth of the third carpal bone was not significantly different between foals of 5 and 11 months old (Firth et al., 1999b). BMDv was determined in the SCB of the proximal articular surface of the proximal phalangeal bone of these six groups (three exercise regimens, two ages) at 1-, 2-, 3-, 4- and 5-mm depth from the articular surface at two differently loaded anatomical sites (site 1 at the proximo-dorsal eminence and site 2 at the middle of the medial articular surface; site 2 is continuously loaded, site 1 intermittently). At 5 months BMDv was significantly higher at site 2 than 1 with highest values and most apparent difference at depths to 3 mm. By 11 months, site differences were less, due to a greater increase in BMDv at site 1 in the previous 6 months, and BMDv was significantly higher in site 2 than 1 in previously boxed/sprinted and trained groups but not previously pastured foals. The significantly higher BMDv in pasture and boxed/sprinted than boxed foals at 5 months was no longer present at 11 months at site 1, and at site 2 the previously boxed/sprinted foals had significantly higher BMD than previously boxed and pastured groups. Continuous loading in the central area of the metacarpophalangeal joint surface, which is present even during standing and slow gaits, apparently stimulated distinct and significant changes in the SCB to a greater extent than intermittent loading at the proximal eminence of the proximal phalangeal bone (Brama et al., 2009). In the GEXA study 18 of 33 Thoroughbred foals born and raised at pasture began gentle exercise at 3 weeks of age and continued until ~17 months of age. The remaining 15 foals had spontaneous pasture exercise only. The bone parameters of the foals having only spontaneous pasture exercise (PASTEX group) were compared with those of the foals subjected to additional exercise (1030 m per day, 5 days/week; CONDEX group) (Rogers et al., 2008a), by pQCT undertaken serially at average ages of 4 days, and 2, 4, 12 and 17 months (Firth et al., 2010). The mid-diaphysis of the proximal phalangeal and third metacarpal bone increased its resistance to deformation by increasing bone mass, circumference, and mean cortical thickness. The imposed exercise resulted in highly significant differences in these parameters when serial values over time were pooled, but were significant at specific ages (12 and 17 months) only in the phalangeal bone. There was no significant difference between PASTEX and CONDEX groups with regard to cortical bone density, indicating that the increase in bone strength is related to a far greater extent to increase in bone size and mineral content or to other factors such as collagen characteristics (see above) than to change in BMDv. In the proximal metaphysis, the trabecular BMDv (inner 45% of the scan slice) was lower in the CONDEX than PASTEX group at 2 months of age and over the whole 17 months when values were pooled, most likely associated with the bone area of the CONDEX group being greater, although not significantly. In the outer ring of the metaphysis, consisting of both trabecular and cortical bone, bone mineral content was significantly greater over time in CONDEX than PASTEX; bone area was also greater but not significantly. The interpretation is that the increase in size and mineral content of the outer part of the metaphysis made the bone stiffer, resulting in lower strain and less mineral accretion response in the central part of the metaphysis of the CONDEX group. The conditioning exercise from 3 weeks to 17 months of age appeared to have no negative effects on bone, since no clinical radiographic abnormalities were detected. Also, the reduced bone density in the sagittal groove detected by CT (Kawcak et al., 2009) and previously described (Doube et al., 2007) were present in both PASTEX and CONDEX foals. Point projection radiography (Firth et al., 2009) of the same joints of both conditioned and non-conditioned horses showed osteochondrosis lesions at the disto-dorsal aspect of the sagittal ridge, which apparently heal spontaneously without negative effect on subsequent athletic activity (Kane et al., 2003). This supported previous indications (Barneveld & van Weeren, 1999; van Weeren & Barneveld, 1999b) that the presence and severity of osteochondrosis is not affected by exercise in the young horse. In nineteen of the GEXA trial horses the bone response to race training and withdrawal from training was determined (Firth et al., 2007; Firth et al., 2012) by pQCT scanning immediately before training began, after training stopped, and after the horses had been at pasture for some months. In the diaphysis, the density of the third metacarpal bone was approximately 0.8% higher at the end of 2-year-old training than before it started, but it had returned to the previous value after being at pasture for several months. During training, diaphyseal bone strength index (the resistance of the bone to bending and torsion) increased, due to both the slight density increase but much more to the increase in size of the bone; the strength index continued to increase after pasturing, most likely because the horses were still growing, although the independent effect of the training exercise itself on diaphyseal size increase could not be tested. In the epiphysis, there was no increase in cross-sectional area, but substantial and significant increase in mineral content and BMDv. In the inner zone of the epiphysis, trabecular bone density increased, as did density of the outer zone consisting of trabecular and more compact bone fractions; in neither did withdrawal from exercise for several months at pasture result in significant decrease in BMDv. In 19-month-old Thoroughbred fillies, which had been treadmill exercised for 18 weeks, bone density of the distal radius, radial carpal bone, third carpal bone and proximal third metacarpal bone was significantly greater than in age-matched horses that had been given only walker exercise (Firth et al., 1999b). The adaptive changes in the trabecular bone was very obviously localized to the load path of the higher forces resulting from the higher speed exercise, and resembled those found in horses that had been exercised for 19 months in an earlier experiment (Firth et al., 1999c). Some of these changes were discernible after 13 weeks of early training (Fig. 13.11). Difference in bone density and in radionuclide uptake in carpal bones was not detected between 2-year-old horses exercised on a treadmill 5 days per week for 6 months and control horses walked for 7 min daily; that there were differences in the fetlock joints shows not only the specific adaptive response within bones, but also a regional difference in the response of different bones to the exercise imposed upon the animal (Kawcak et al., 2000). Recently, study of carpal bone tissue from Thoroughbreds aged 3–22 years showed that increased bone formation was accompanied by increased bone collagen synthesis and remodeling, particularly within the trabecular regions of the bone of horses in racing compared to those with no such history. The increase in bone density would lead to greater stiffness, particularly in the ‘stiffer’ cortical bone, the failure of which was suggested to be associated with reduced support from the weaker underlying trabecular bone because of lower mature cross-link content (Tidswell et al., 2008). These findings and their suggested significance, in terms of end-stage disease that supervenes in racehorses (Pool, 1996), remain unclear at this time. Fig 13.11 Radiograph of an identical preparation to that in Figure 13.10 from a 19-month-old pasture-raised Thoroughbred filly that underwent 13 weeks of early training for racing. The orientation of the metaphyseal and epiphyseal trabecular bone is different from that in the untrained horse in Fig. 13.10, there is increased radiodensity in the dorsal load path in the intermediate carpal and radial epiphysis, and the subchondral bone line is regionally thickened. The dorsal cortical shell of the radial epiphysis and intermediate carpal bone is thicker. The changes are typical of the general changes present after galloping exercise. Horses aged 22 months trained conventionally on grass and sand racetrack surfaces were compared with horses kept in large grass yards for 13 weeks. Four horses completed the training program, and three completed only cantering exercise and did almost no galloping (Firth et al., 2004). The latter had Mc3 diaphyseal bone CSA, mineral content, periosteal circumference, and bone strength similar to untrained horses, but less than those horses that had galloped. However, cantering exercise had not caused growth of the bone shaft, and cortical BMDv of horses that had cantered only was similar to that of galloping horses, both being significantly higher than that in untrained horses, despite numbers being small (Firth et al., 2005). The results were consistent with the previous studies in which metacarpal cortical thickness increased in treadmill-trained yearlings that had galloped (McCarthy & Jeffcott, 1992). Significant relationships existed between months in training and Mc3 bone geometry and moment of inertia in 2–4-year-old racehorses (Sherman et al., 1995). It was further shown that lunging yearling Quarter Horses for 20 min/day for 8 weeks had no significant effect on osteocalcin concentrations (Fenton et al., 1999), and that velocities >12 m/s were required to affect the Mc3 dorsal cortex dimensions (Davies et al., 1999). In the Mc3 epiphysis, early training resulted in highly localized BMDv increases, with different patterns to that in Mt3 (Firth et al., 2005), which was described in detail (Boyde & Firth, 2005). The changes in the galloped horses resembled those found in the proximal sesamoid bone of 2-year-old racehorses, in which 5 months of training on dirt tracks was associated with significantly higher densities and greater trabecular width compared with horses at pasture (Young et al., 1991b). Similar regional adaptive changes were present in the medial facet of the third carpal bone, in which the remodeling response of the SCB was not uniform between horses, with higher densities being present in those that had galloped least. The bulk trabecular bone apparently responded by formation of increased bone mass in an arrangement resembling pillar structures deep to the SCB (Firth & Rogers, 2005). Such changes are consistent with indentation studies in the dorsal aspect of third carpal bones of 2- and 3-year-old Thoroughbred horses that had raced or trained, which had higher density and stiffness of the third carpal bone than did untrained horses. Area fraction and stiffness were closely related, and were higher in horses with than without pathological change (Young et al., 1991a). Lastly, in this cohort of 2-year-old horses, changes in subchondral bone were detected in subchondral bone and articular calcified cartilage of the distal Mc3. The lesions were present in both trained and untrained horses, and it was suggested that the forces associated with athletic training might be less important than factors related to bone development (Boyde & Firth, 2008). Although it is impossible to quantify the etiological significance of either, the findings are mentioned here to reinforce the point already alluded to above under cartilage, namely that optimal development requires a certain biomechanical environment to proceed optimally, and inappropriate exercise imposed early or later in juvenile life, can negatively affect bone development. The age at which horses are considered adult remains less than definitive, but if adulthood is accepted as being around the end of the horse’s fourth year, there are almost no studies of the effect of exercise on bone of mature horses, besides studies of cumulative or excessive exercise loads resulting in pathology and/or clinical signs which are not considered in this text. There remains difficulty in assessing the effect of exercise since much of the adult response most likely is influenced by adaptive changes in earlier years, the nature of which will vary between individuals. This explains why the little information on adaptive responses to exercise in adult horses is confined to cross-sectional information, such as that concerning carpal change (see above) and radial diaphyseal BMDv (Riggs, 1993b). The horse’s skeletal musculature is highly developed, particularly in athletic breeds. In contrast to most mammals, in which 30–40% of bodyweight consists of muscle, and to non-athletic horse breeds (~42%), more than half (~55%) of a mature Thoroughbred’s body weight comprises skeletal muscle (Gunn, 1987). Low body fat and a large amount of muscle likely reflect adaptation and selective breeding of animals used for elite endurance and sprint racing (Kearns et al., 2002). Total muscle blood flow in horses that are exercising at a level when O2 consumption is at its maximum (VO2max, 134 ± 2 mL/min/kg) has been estimated at 226 L/min, which represents approximately 78% of total cardiac output (Armstrong et al., 1992). During exercise and locomotion metabolites and oxygen reach skeletal muscle fibers via the respiratory, cardiovascular, and hematologic systems; in turn the muscle fibers produce energy in the form of adenosine triphosphate (ATP), which, via the contractile machinery, is converted into mechanical work. The structural arrangement of the musculoskeletal system permits the use of this energy to move the horse’s limbs in a characteristic rhythmic pattern that is well established for each gait. Equine skeletal muscle is relatively heterogeneous and has considerable potential to adapt during training, largely mediated by the structural and functional plasticity of muscle fibers. These long-term (weeks to months) adaptations occur independently from the immediate or short-term muscular metabolic responses to either a single bout of sub-maximal or near-maximal exercise. They are associated with altered rates and regulation of transcription of specific genes and consequently a change in the amount of specific isoforms of proteins expressed within individual fibers (Williams & Neufer, 1996). Depending on the basal muscle status (e.g. breed, age, sex, level of fitness and training history of the horse), and characteristics of the stimulus (e.g. nature, intensity, duration, and frequency of exercise bouts, and total length of the conditioning program), the adaptive response to training can take two different forms (Table 13.1). First, the quantitative responses, when myofibers increase in size (hypertrophy) but otherwise retain their basal structural, physiological, and biochemical properties. And secondly, the qualitative responses or remodeling without hypertrophy, where myofibers do not change in size but acquire markedly different enzymatic and structural characteristics (e.g. fiber type transitions). In practice, the most common adaptive responses of equine skeletal muscle to training is a mixed form of remodeling with discrete or substantive hypertrophy, that is often accompanied by increases in the number of capillaries (see Rivero, 2007; Rivero & Piercy, 2008). As already mentioned, the modality and amplitude of the muscular responses to training also depend significantly on the muscle profile before training (Pette & Staron, 1997) because the increased contractile activity that is associated with training always induces a change toward slow and oxidative muscle profiles. Hence fast-twitch fibers (and therefore muscles that contain a higher proportion of fast-glycolytic fibers) can show a relatively greater training adaptation than slow-twitch fibers. As a general rule, this response is particularly prominent in young, inactive (Yamano et al, 2002) and aged, sedentary (Kim et al, 2005) horses, which have a high percentage of glycolytic (low oxidative) type IIX fibers (Fig. 13.12A,B), in contrast to active mature horses, which have muscle fiber type profiles that are more oxidative (Fig. 13.12C,D). Although it can be hard to differentiate muscular adaptations caused by growth and training, specific training effects have been delineated in growing foals (Dingboom et al, 2002; Eto et al, 2003; Rietbroek et al, 2007a,b), and young horses (Essén-Gustavsson et al, 1983; Ronéus et al. 1992, 1994; Rivero & Serrano, 1999; Serrano & Rivero, 2000; Serrano et al, 2000; Yamano et al, 2002).
The response of musculoskeletal tissues to exercise
General introduction
Articular cartilage
Introduction
Basic principles of articular cartilage biology
Developmental aspects of articular cartilage
The effect of exercise on articular cartilage during growth and development
The effect of exercise on articular cartilage in young adult animals
Conclusion
Bone
Introduction
Morphology and physiology of bones
General response of bone to exercise
The need for exercise
The response of bone to exercise in the horse
Muscles
Overview
The response of musculoskeletal tissues to exercise
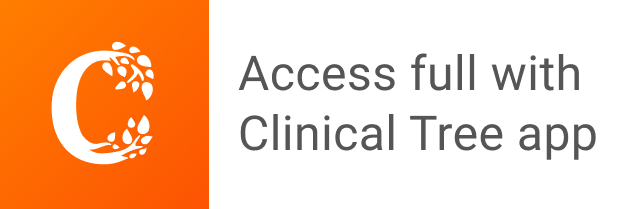