CHAPTER 9 Importance of the respiratory system in the athletic horse During the last several decades, research in exercising horses provided growing evidence that the respiratory system may be a limiting factor for maximal performance, even in healthy animals. (Art et al., 1990a; Bayly et al., 1983; Bayly et al., 1987; Erickson et al., 1991; Hodgson et al., 1990; Jones and Lindsdtedt, 1993; Knight et al., 1991; Poole, 2004; Thornton et al., 1983; Wagner et al., 1989). Therefore, any pulmonary dysfunction, even subclinical or moderate, may significantly impair the aerobic metabolism of exercising horses. The observation that respiratory abnormalities frequently are responsible for the “poor performance” syndrome in the horse (Arthur, 1990; Evans and Marlin, 1999; Franklin et al., 2012; McNamara et al., 1990; Morris and Seeherman, 1991) confirms the importance of optimal pulmonary function in the athletic horse. A good understanding of the peculiarities of equine respiratory structure and function is essential for a comprehensive evaluation of the respiratory system and possible correction of its dysfunction. If the respiratory system is able to influence some nonrespiratory functions, the opposite is also true. Pulmonary function may be disturbed by factors that are not directly related to the integrity of the respiratory system such as the quality of the inspired air, the position of the head and neck, the abdominal mass, locomotion-respiration coupling, cardiac function, the equipment used, and a range of other factors. All these factors must be taken into account when evaluating equine pulmonary function. The function of an organ is highly influenced by its anatomy, and vice versa. This is particularly true with regard to the respiratory system, where external respiration induces important structural changes, mainly during exercise-induced hyperpnea. It is, therefore, useful to remember some structural peculiarities that do influence pulmonary function in the athletic horse. More morphologic details can be obtained from relevant anatomy handbooks (e.g., Hare, 1975). At a functional level, the most important structural peculiarity of the equine upper airways is the intrapharyngeal ostium, which is an opening in the soft palate formed caudodorsally by the palatopharyngeal wall, laterally by the pillars of the soft palate, and rostrally by the visible border of the soft palate. The laryngeal structures, that is, the corniculate cartilages and the epiglottis, articulate with the ostium like a button in a button hole (Cook, 1989) forming an airtight seal when the horse breathes (Figure 9-1). This peculiar arrangement explains why the horse is an obligatory nasal breather. Indeed, because of this anatomic characteristic, the horse, unlike humans and dogs, is not able to switch from nasal breathing to oronasal breathing when the nasal resistance to airflow becomes too high, as occurs during exercise-induced hyperpnea. In horses, the displacement of the caudal border of the soft palate to a position above the epiglottis, called dorsal displacement of the soft palate, is not physiologic, except when it occurs during swallowing, coughing, or whinnying (see Figure 9-1). In all other conditions, this dorsal displacement is abnormal and will induce dyspnea, especially during strenuous exercise. It induces a narrowing of the upper airways and causes a soft palate flapping, sometimes resulting in a dramatic asphyxia in racing horses. In some parts of the world, this dysfunction is called “choking down.” The structural and functional peculiarities of the equine larynx make it a potential bottleneck in the upper airways, that is, the narrowest portion of the upper airway. The rostral protrusion of the laryngeal cartilages through the intrapharyngeal ostium constitutes the aditus laryngis. This pharyngeal opening is formed dorsally by the corniculate cartilages, laterally by the vocal folds, and ventrally by the epiglottis (Figure 9-2). The aditus laryngis can vary from tight seal, that is, full adduction of the laryngeal structures during swallowing to protect the lower airways from ingesta, to a maximal opening, that is, full abduction of these structures during exercise-induced hyperpnea to decrease the resistance to air flow (see Figure 9-2). This full opening is completed by a dilation of the larynx caused by contraction of the intrinsic muscles, which eliminates the opening of the laryngeal ventricles. Any impairment of this laryngeal dilatation due to structural factors (such as rostral displacement of the palatopharyngeal arch) or functional factors (such as laryngeal hemiparesis) will be responsible for inadequate ventilation during heavy exercise (Bayly et al., 1984; Franklin et al., 2012) and will generally induce abnormal respiratory noises related to increased air flow resistance (see Figure 9-2). The horse’s trachea is a 70- to 80-cm-long flexible tube consisting of 48 to 60 cartilaginous rings that are open dorsally (Figure 9-3). The free ends of these plates overlap in the cervical part, but not in the thoracic part, of the trachea (Hare, 1975). Its cross-section is almost circular proximally and distally (transverse diameter 5.5 cm; sagittal diameter 5 cm) but is more flattened dorsoventrally (transverse diameter 7 cm, sagittal diameter 5 cm) between the two extremes. Routine endoscopic evaluations of the lower airways of horses examined for exercise intolerance show evidence that there is sometimes a sharp lateral narrowing of the intrathoracic trachea just cranial to the tracheal bifurcation. Despite its cartilaginous structure, the extrathoracic trachea is quite compliant and is susceptible to collapse during the highly compressing transmural pressure that occurs during forced inspiration (Art and Lekeux, 1991a; Franklin et al., 2012). Its compliance (and, therefore, its collapsibility) is significantly decreased, however, by smooth muscle contraction (due to exercise-induced adrenal discharge) and by tracheal extension (due to exercise-induced head and neck stretching). Moreover, the resistance to collapse is also dependent on the shape of the tracheal cross-section. In this regard, all horses do not seem to be equal. Indeed, the transverse to sagittal diameter ratio varies from 0.9 (i.e., circular shape) to 2 (i.e., an elliptical shape) with a mean of 1.4 (Art and Lekeux, 1991b) (Figure 9-4). The more ellipsoid the trachea, the more compressible it is. Therefore, horses with a transverse to sagittal diameter ratio higher than 1.5 are disadvantaged and more susceptible to exercise intolerance caused by insufficient ventilation. The size and age of the horse do not influence the compliance of the trachea (Art and Lekeux, 1991b; Franklin et al., 2012). After the bifurcation of the trachea into the right and left principal bronchi, the bronchial tree branches many times to the periphery of the lung via the primary bronchi, the segmental bronchi, the bronchioles, and the terminal bronchioles (Figure 9-5). These intrathoracic conducting airways are also susceptible to collapse when the transmural pressure exerted on their walls is compressive. This is particularly true at the level of the small airways, which do not have cartilaginous support. However, in contrast with extrathoracic airways, where partial dynamic collapse occurs only during inspiration, the collapse of small airways occurs only during forced expiration, that is, when the extraluminal pressure is more positive than the intraluminal pressure (Figure 9-6). Respiratory bronchioles are poorly developed in the horse, and most of the gas exchange occurs at the alveolarcapillary unit, which is well designed in this species. The horse’s lung is highly developed and represents about 1% of the body weight. It contains at least 107 alveoli and probably 1000 times more capillary segments. The alveolar surface density is large and alveolar septa thin compared with other mammals (Gehr and Erni, 1980). Although the equine lung is not really divided by fissures into lobes, an apical lobe, a diaphragmatic lobe, and an accessory lobe in the right lung and an apical lobe and a diaphragmatic lobe in the left lung are usually described (see Figure 9-5) The lungs are covered by a thick pleura. The connective tissue septa between lobules are not complete. This allows some collateral ventilation, that is, transfer of air between adjacent lobules via accessory pathways such as the interalveolar pores of Kohn, the canals of Lambert, and communicating respiratory bronchioles and alveolar ducts. The advantage of this collateral ventilation is to partially compensate for reduced ventilation in areas with small airway obstructive diseases (Robinson, 1982). However, in horses, these accessory pathways present a high resistance to air flow and are able to provide only a maximum of 16% of the required volume, a very small proportion compared with the 90% recorded in the human lung (Franklin et al., 2012). Therefore, these pathways are probably of limited value for equine pulmonary function, except possibly for the prevention of atelectasis in horses suffering from airway obstruction. The lung receives blood from two circulations (Table 9-1). The pulmonary circulation receives the total cardiac output from the right side of the heart. The branches of the pulmonary artery carry venous blood to the lung, accompany the bronchi, and form rich capillary plexuses on the walls of the alveoli. Here the blood is arterialized (oxygenation and release of carbon dioxide are two main functions) and returned to the left side of the heart by the pulmonary veins. The equine pulmonary arteries adjacent to the bronchioles and the alveolar ducts are muscular and have a rather thick medial smooth muscle layer (thinner than the cow and pig but thicker than the dog and sheep). This amount of smooth muscle determines the reactivity of the vessels to hypoxia and thus explains why a horse may present with pulmonary hypertension caused by a hypoxic vasoconstriction (Bisgard et al., 1975). At the level of the terminal bronchioles, pulmonary and bronchial circulations anastomose. Most of these anastomoses occur at the level of the capillaries and veins rather than the arteries (Magno and Fishman, 1982; McLaughlin, 1983). Compared with the dorsoventrally flattened thorax of the human, the horse has a rather rounded thorax. Moreover, cranially, its thorax is laterally compressed to facilitate locomotor function. The differences in size, shape, and position of the abdomen in relation to the lungs, as well as the high rigidity of the equine thorax (Leith, Gillespie, 1971), are probably advantageous for locomotion and for stabilization of the relaxation volume of the respiratory system during postural changes. Inspiratory muscles: The diaphragm is the main inspiratory muscle. It is a domed musculotendinous sheet separating the abdomen and the thorax. It consists of a costal portion, arising from the xyphoid process and the costochondral junctions of the eighth rib to the fourteenth rib, and a crural portion, arising from the ventral surface of the first several lumbar vertebrae and extending toward the tendinous center of the diaphragm. The apex of the dome extends to the eighth intercostal space at the level of the base of the heart. The external intercostal muscles, which join the ribs, are also active during inspiration. However, during exercise, the serratus ventralis participates in inspiration much more than do the external intercostal muscles (Manohar, 1991). Other inspiratory muscles include those connecting the sternum or the ribs and the head (sternomandibularis, scalenus). When they contract, they pull the sternum or the ribs forward and participate consequently in enlargement of the thorax (De Troyer and Loring, 1986). Expiratory muscles: The abdominal muscles (external and oblique abdominis, transverse and rectus abdominis, transverse thoracis) and the internal intercostal muscles are expiratory muscles. When they contract, they increase the abdominal pressure, forcing the relaxed diaphragm forward and reducing the thoracic volume (De Troyer and Loring, 1986). Other respiratory muscles: Some muscles such as the abductor muscles, which dilate the nares, the pharynx, and the larynx, are able to modify the size of the airways. Innervation: The phrenic nerves, which innervate the diaphragm, come from the cervical spinal cord and traverse down both sides of the heart. In horses suffering from electrolyte imbalances (e.g., after prolonged exercise or diarrhea), the phrenic nerve may become hyperexcitable, and sometimes a diaphragmatic flutter (“thumps”) may be observed (i.e., diaphragmatic contraction in phase with cardiac depolarization) (Refer to Chapter 8). This is usually transient but often responds to intravenous administration of polyionic replacement fluids augmented with calcium borogluconate. The laryngeal muscles are innervated by the recurrent laryngeal nerves. The recurrent laryngeal nerve is one of the longest nerves of the body. The left laryngeal nerve originates from the brain and travels down the neck into the chest as a component of the vagus nerve. At the level of the heart, the recurrent laryngeal nerve branches off the vagus and becomes an individual nerve, which travels back up the neck before finally reaching the larynx. The high incidence of left laryngeal hemiplegia in the horse has been related to neural injuries because of the specific course of the left laryngeal nerve. However, this theory may be challenged by the occasional occurrence of right hemiplegia (Franklin et al., 2012). Expired minute volume: Equine lung volumes are illustrated in Figure 9-7. The volume of air inhaled or exhaled during a normal breath is termed the tidal volume (Curtis et al., 2006; Gallivan et al., 1989a; Ramseyer et al., 2010). Its value in the healthy resting athletic horse is about 12 milliliters per kilogram (mL/kg of body weight. Multiplying the tidal volume by the respiratory frequency gives the expired minute ventilation. Exercise imposes a potent stress on the ventilatory pump: As speed increases, minute ventilation increases almost linearly, and the expired minute ventilation, which averages 80 liters per minute (L/min) at rest (Table 9-2), may reach values in the vicinity of 1800 L/min during heavy exercise (Art and Lekeux, 1993; Art and Lekeux, 1995; Katz et al., 2005; Knight et al., 1991). Indeed, this may be an underestimation. The change in minute ventilation necessary to meet the gas exchange requirements can be reached by changing tidal volume, respiratory frequency, or both. TABLE 9–2 Mean Respiratory Values in Healthy Thoroughbred Horses Considered Average for Their Quality, Fitness, Age (5 Years), and Size (Body Weight 470 kg) and Running on a Treadmill under Temperate Climatic Conditions (Lung and Gas Volumes are Expressed as BTPS and STPD Respectively). * †Pp1min: peak intrapleural pressure recorded during inspiration; Ppl max: peak intrapleural pressure recorded during expiration; maxΔPpl: maximum change in intrapleural pressure; Pin: inertial pressure; ΔP ∞ §HR: heart rate; SV: stroke volume; In horses, when trotting, the increase in minute ventilation is achieved by a simultaneous increase in tidal volume and respiratory frequency at low exercise intensities and mainly by an increase in respiratory frequency at high exercise intensities (Art and Lekeux, 1988a; Curtis et al., 2006; Evans and Marlin, 1999). Values as high as 133 breaths/min have been reported in Standardbred horses running on a treadmill (Dahl et al., 1987; Franklin et al., 2012). In galloping horses, the respiration and the locomotion are compulsorily coupled (Bramble and Carrier, 1983; Franklin et al., 2012). Step and respiratory frequencies average 110 to 130 per minute with maximum values of 148 per minute reported (Franklin et al., 2012; Hörnicke et al., 1983; Hörnicke et al., 1987). Therefore, when the horse gallops, the increase in minute ventilation with increasing speed is due mainly to the increase in tidal volume rather than in respiratory frequency. Tidal volumes between 12 L and 15 L are reported in fast galloping horses (Art et al., 1990a; Bayly et al., 1987; Franklin et al., 2012; Hörnicke et al., 1987; Landgren et al., 1991). Only a portion of the inspired volume reaches the area of the lung where gas exchange takes place; this is the alveolar ventilation. The remaining part of the minute ventilation is wasted in the regions of lung where no gas exchange occurs; this is the physiologic dead space ventilation, which includes the conducting airways (anatomic dead space) and the alveoli that are ventilated but not perfused (alveolar dead space). The dead space to tidal volume ratio averages 50% to 60% in the resting horse (Curtis et al., 2006; Franklin et al., 2012; Gallivan et al., 1989b; Lekeux et al., 1992), a percentage twice as large as reported in other athletic species such as humans and dogs. For any given minute ventilation, the lower the physiologic dead space ventilation, the higher is the alveolar ventilation and the better is the gas exchange. However, the evidence that adequate gas exchange is maintained with very low tidal volume and very high respiratory frequency (600 breaths/min, i.e., high-frequency or jet ventilation) suggests that such factors as mass convection, convective dispersion, and molecular diffusion may provide an adequate gas transport between the atmospheric air and the alveoli despite a very high dead space to tidal volume ratio (Chang, 1984; Froese and Bryan, 1981). Exercise-induced changes in alveolar ventilation and dead space to tidal volume ratio in horses depend on the type of exercise performed (Bayly et al., 1987; Curtis et al., 2006; Franklin et al., 2012; Pelletier et al., 1987; Pollman and Hörnicke, 1984; Rose and Evans, 1987). During mild-to-moderate exercise, the dead space volume does not change significantly (Curtis et al., 2006; Franklin et al., 2012; Pelletier et al., 1987). Therefore, the increase in tidal volume will increase the alveolar ventilation and decrease the dead space to tidal volume ratio. If the exercise is prolonged at a constant rate, the dead space ventilation will increase by a simultaneous increase in respiratory frequency and in the dead space to tidal volume ratio (Curtis et al., 2006; Franklin et al., 2012; Pelletier et al., 1987; Rose and Evans, 1987). This adaptation probably reflects the thermoregulatory role of the respiratory system (see Chapter 8). Lastly, during intense effort, there is a decrease in this ratio from about 60% to 20% (Curtis et al., 2006; Franklin et al., 2012; Lekeux et al., 1992; Rose and Evans, 1987). In absolute terms, the physiologic dead space is reduced from 3.5 L at rest to 2.5 L during heavy exercise. Because the anatomic dead space averages 2.5 L at rest and is expected to remain unchanged during exercise, the exercise-induced difference in the dead space (l L) is probably attributable to the disappearance of the alveolar dead space (i.e., alveoli that are ventilated but not perfused) induced by the recruitment of previously nonfunctional pulmonary capillaries (Curtis et al., 2006; Franklin et al., 2012; Lekeux et al., 1992). The distribution of ventilation is not uniform in the lung, even in healthy horses. This occurs for two different reasons. The main reason is that the intrapleural pressure changes are not uniform all over the thoracic cage. Because of gravitational effects, pressure is more negative in the dorsal than in the ventral part of the lung (Derksen and Robinson, 1980; Franklin et al., 2012). Consequently, the dorsal alveoli are more distended, less compliant, and receive less air during inspiration at any ventilatory rate. A second reason may be the occurrence of some inequalities in the regional small airways resistance, alveoli compliance, or both; inhaled air preferentially enters the areas of the lungs with low resistive airways and highly compliant alveoli. Figure 9-8 displays how the alveoli that follow highly resistive airways (B), or which have a lower compliance, will fill up much more slowly than the others (A) (Otis et al., 1956). This ventilatory asynchrony is moderate in healthy horses and does not have significant effects on gas exchange at low respiratory frequencies. However, in horses with significant asynchrony (i.e., subclinical small airways disease) and with a high respiratory frequency (i.e., during exercise), this phenomenon will significantly impair gas exchange and may result in poor performance (Curtis et al., 2006; Derksen et al., 1992; Franklin et al., 2012) (see Figure 9-8). The interdependence between adjacent lung regions tends to limit the nonuniform changes in regional ventilation (Franklin et al., 2012; Robinson and Sorenson, 1978). These mechanical interactions are the result of the intricate mesh of interconnecting elastic and collagenous tissue fibers in the lung. Collateral ventilation between adjacent lung areas is also potentially able to reduce the nonuniformity of the ventilation distribution. However, in the horse, these collateral pathways, because of their high resistance to air flow, are of limited usefulness at low respiratory frequencies and probably of no functional importance at all at high respiratory frequencies (Robinson and Sorenson, 1978). All factors that either interfere with the properties of the lungs, such as decrease in the compliance of the alveoli (interstitial edema), increase in the resistance of small airways (collapse, bronchospasm, hypersecretion, blood), or increase in respiratory frequency (during exercise), are likely to increase the ventilatory asynchrony and impair the gas exchange. Exercise, by increasing the respiratory frequency, probably magnifies the regional differences in ventilation (see Figure 9-8). The lobules, which have a long time constant for filling, do not fill adequately before expiration begins, and consequently, ventilation–perfusion mismatching and hypoxemia result. Moreover, because of the interdependence between adjacent lung areas, the lobules with increased airflow resistance, decreased compliance, or both are stretched and compressed by the surrounding lung parts (Mead et al., 1970; Franklin et al., 2012). This may induce abnormal stresses on the tissues of these lobules. It has been suggested that exercise-induced pulmonary hemorrhage could sometimes be a consequence of this pulmonary overstretching (Robinson, 1979). The differences between pulmonary and bronchial circulations are outlined in Table 9-1. Pulmonary vascular resistance is very low; it is estimated at only one seventh the resistance of the systemic circulation. Consequently, the mean pressure throughout these vessels is much less than in the systemic circulation (∼25 versus ∼125 mm Hg). Although the vascular resistance in the systemic circulation is totally due to the precapillary vessels, the pulmonary vascular resistance is equally distributed between the pre- and postcapillary vessels. Because of the peculiar distribution and the low value of the pulmonary vascular resistance, pressure in the pulmonary capillaries remains pulsatile. During strenuous exercise, pulmonary blood flow increases five- to eightfold (Franklin et al., 2012; Parks and Manohar, 1983; Thomas and Fregin, 1981; Thomas et al., 1983; Thornton et al., 1983). A marked simultaneous pulmonary hypertension is a feature of exertion in horses and ponies; the mean pulmonary arterial pressure rises about threefold, from 28 mm Hg at rest to about 84 mm Hg at a fast gallop, with maximal reported values of 100 mm Hg (Erickson et al., 1990; Erickson et al., 1992; Evans and Rose, 1988a, b, c; Franklin et al., 2012; Goetz and Manohar, 1986; Wagner et al., 1989). The pulmonary right-to-left shunt of the cardiac output is approximately 1% at rest. It may decrease up to 0.4% during heavy exercise (Franklin et al., 2012; Wagner et al, 1989). Factors capable of modifying the pulmonary vascular resistance are either extravascular or intravascular. Changes in lung volume represent the main extravascular factor. The pulmonary vascular resistance increases at extreme lung volumes because of the compression of the lung vessels during forced expiration (small lung volume) or during forced inspiration (lung distended) (Fishman, 1985). The increase in blood viscosity related to the exercise-induced rise in packed cell volume is another extravascular factor influencing pulmonary vascular resistance; each increase of 1% in the packed cell volume induces an increase of 4% in the pulmonary vascular resistance (Taylor et al., 1989). Vasoactive compounds or changes in the local composition of blood are intravascular factors that regulate the pulmonary vascular resistance by modifying vascular smooth muscle tone. Pulmonary vascular resistance decreases with an increase in blood flow, pulmonary arterial pressure, or both (Franklin et al., 2012; Taylor et al., 1989). This results from a combination of dilation of the perfused vessels (increase in their cross-sectional area and consequently decrease in their flow resistance) and recruitment of previously unperfused vessels. Therefore, despite the substantial increase in packed cell volume, there is an approximately threefold decrease (from 0.32 to 0.11 mm Hg/L/min) in pulmonary vascular resistance with strenuous exercise (see Table 9-2). Gravitational factors: It has been shown that there is a vertical gradient of pulmonary blood flow, with the ventral regions receiving more perfusion per unit lung volume than the dorsal regions (Amis et al., 1984; Franklin et al., 2012). According to the relative magnitudes of pulmonary arterial, venous, and alveolar pressures, blood flow in the lung can be divided into four zones (Fishman, 1985). In zone 1, at the top of the lung, there is no blood flow, because the mean pulmonary arterial pressure is too low to overcome the hydrostatic pressure imposed by the column of blood connecting the pulmonary artery to the apical blood vessels. Therefore, alveolar pressure exceeds both pulmonary arterial and venous pressures, and the collapsible capillaries remain closed. However, because the mean pulmonary arterial pressure is about 15 to 18 mm Hg (i.e., 20 to 25 cmH2O), it may be sufficient to perfuse the vertical height of the lung above the heart. This zone is probably small in most horses. Because this lung region is unperfused, it does not participate in gas exchange and represents the “alveolar dead space.” During exercise, the increased pulmonary arterial pressure probably improves the recruitment of the vessels in zone 1 and therefore makes the distribution of perfusion more homogeneous. The alveolar dead space is estimated to be 0.8 L to 1 L in the resting horse or about 3.5% of the functional residual capacity and is very likely to disappear in the exercising horse (Franklin et al., 2012; Lekeux et al., 1992). In zone 3, both pulmonary arterial and venous pressures exceed alveolar pressure; capillaries are perfused throughout their length and are increasingly distended down this zone. A zone 4 is sometimes described in which the pulmonary blood flow decreases as a result of a compressing interstitial pressure on the vessel. Although it is well established that pulmonary blood flow is distributed in a vertical direction with respect to gravity, Hakim et al. (1987) suggested that in humans there also could be a gradient from the center to the periphery. Because local or peripheral vascular resistance rises in proportion to distance from the lung hilum, the center of each lobe will be better perfused than its periphery. Humoral and neural factors: Although predominantly passive mechanical forces determine regional blood flow distribution, the smooth muscles in arteries and veins respond to vasoactive compounds. The magnitude and mechanism of these responses depend on numerous factors such as the pre-existing level of pulmonary vascular tone and the integrity of the pulmonary vascular endothelium (Bray and Anderson, 1991). Hypoxic vasoconstriction: The modification of ventilation in some regions of the lung or in the whole lung also influences pulmonary perfusion. In unventilated regions of the lung, alveolar hypoxia occurs, inducing a local hypoxic vasoconstriction. This constriction provides a mechanism to redistribute pulmonary blood flow from less-ventilated regions to well-ventilated regions and therefore improves the ventilation to perfusion ratio and the gas exchange. The magnitude of the response to hypoxia depends on the thickness of the pulmonary arterial smooth muscle layer; the response of the horse is intermediate between such species as cattle and pigs, in which the response is quite vigorous, and sheep and dogs, in which the response is minimal (Bisgard et al., 1975; Robinson, 1982). The mechanism for the occurrence of this constriction is still unclear. A combination of the action of a vasoactive agent with cellular mechanisms has been suggested as a possible cause. Hypoxic vasoconstriction is generally advantageous when occurring locally but may become unfavorable when occurring through the whole lung, as occurs in acute hypoxia. Exercise-induced pulmonary hypertension occurring in the horse does not appear to be caused by hypoxic pulmonary vasoconstriction (Franklin et al., 2012; Pelletier and Leith, 1991). The bronchial circulation receives approximately 1% to 2% of the cardiac output from the left side of the heart (i.e., oxygenated blood). It supplies the airways, large pulmonary blood vessels, septa, pleura, and other lung structures. However, the lung does not suffer from a partial obstruction of the bronchial circulation. The numerous anastomoses between the pulmonary and bronchial circulations may provide blood flow to the bronchial circulation (Franklin et al., 2012; Taylor et al., 1989). Conversely, when the pulmonary perfusion is locally impaired, the bronchial circulation may proliferate and maintain some blood flow throughout the lung, thus contributing partly to gas exchange (Lilker and Nagy, 1975). Such vascular proliferations also may occur in response to pulmonary inflammation. Because these may be extensive in the dorsocaudal regions of the lungs, which are the regions that sometimes bleed during intense exercise, they have been related to the occurrence of exercise-induced pulmonary hemorrhage. In addition, the role played by pulmonary hypertension, bronchial hypertension, or both in exercise-induced pulmonary hemorrhage is strongly suspected but has not been demonstrated so far (Franklin et al., 2012). Postpulmonary shunts may result from anastomoses between pulmonary and bronchial circulations and, theoretically, may be partly responsible for the fall in the arterial oxygen partial pressure (pO2) observed during exercise. However, a shunt of 1%, which is a reasonable approximation, would reduce arterial pO2 by 5 mm Hg, whereas the actual decrease during exercise is much larger (Franklin et al., 2012; Wagner et al., 1989). Fluid filtration occurs between the capillaries and the interstitial tissue. The alveolar endothelium is less permeable than the capillary endothelium. Therefore, the fluid does not leak into the alveoli unless the epithelium is damaged or unless there is a considerable accumulation of fluid in the interstitial tissue. Fluid filtered from the capillaries moves through the interstitium toward perivascular and peribronchial tissues, where lymphatic vessels are located. During exercise, the marked increase in the pulmonary arterial pressure probably induces an increase in the rate of fluid filtration across the capillary walls of the lung. The fact that this condition is not associated with pulmonary edema suggests that in horses, as in other species (Coates et al., 1984), the lymphatic system has a substantial capacity to drain the pulmonary interstitial space (Taylor et al., 1989). Moreover, the “pumping” action associated with the large and frequent pressure changes in the equine thoracic cage during exercise could contribute to the adequacy of the lymphatic drainage. Most lung regions have a ventilation to perfusion ratio of 0.8, but regions exist that are excessively perfused (ventilation/perfusion ratio <0.8) or ventilated (ventilation to perfusion ratio >0.8). When ventilation is impaired (i.e., partial airway obstruction), the ventilation to perfusion ratio decreases, and in extreme cases of total airway obstruction, the ventilation to perfusion ratio is 0. This corresponds to a right-to-left shunt. If perfusion is inadequate (because of pulmonary vasoconstriction or hypotension) the ventilation to perfusion ratio will increase, and in extreme cases of underperfusion caused emboli or in zone 1, the ventilation to perfusion ratio becomes infinite (Figure 9-9). In quiet, resting horses, the ventilation to perfusion ratio is not influenced by the gravity; that is, it is uniform from the top to the bottom of the lung, suggesting that the gradient in lung ventilation is matched by the gradient in lung perfusion (Amis et al., 1984). In horses exercising heavily, there is only a very slight mismatch of ventilation and perfusion. This mismatch accounts for 25% of the increase in the alveolar–arterial pressure difference in O2 (Franklin et al., 2012; Wagner et al., 1989). The physiologic characteristics of the ventilation to perfusion ratio of horses contrast with those in humans. In the latter, the ventilation to perfusion ratio is nonuniform at rest, and a true mismatching occurs during exercise (Franklin et al., 2012; Gale et al., 1985). In subjects suffering from airway disease, exercise will enhance ventilation–perfusion mismatching, mainly the result of impairment of ventilation. This is particularly true in horses, in which the collateral ventilation has little ability to compensate for the nonpermeability of small airways (Robinson and Sorenson, 1978). Therefore, the lobules that take a longer time to fill will have an inadequate ventilation to perfusion ratio with resultant hypoxemia and, sometimes, hypercapnia. Composition of the respiratory gases The ambient pO2, that is, the relative contribution of the O2 pressure to the total pressure of the ambient gas mixture (air), is about 20.93% of the total pressure exerted by air (air has a pressure of 760 mm Hg at sea level). The partial pressure of CO2 in ambient air is negligible (0.03%) (Figure 9-10). After passing through the nasal cavities and the upper airways, air is saturated with water (H2O). At near normal body temperature (37°C), the partial pressure of the water vapor represents about 6.1% of the total pressure, or 47 mm Hg. Therefore, the other gases exert a total pressure of only 713 mm Hg (or 760 mm Hg minus 47 mm Hg). Therefore, the actual O2 pressure of air in the trachea decreases from 159 mm Hg (room air) to 149 mm Hg.
The respiratory system: Anatomy, physiology, and adaptations to exercise and training
Introduction
External factors influencing pulmonary function
Structural peculiarities of the equine respiratory system and their functional impact
Airways
Pharynx
Larynx
Trachea
Bronchi
Lungs
Blood supply
Thoracic cavity
Respiratory muscles and their innervation
Functional peculiarities of the equine respiratory system at rest and adaptations during exercise
Ventilation
o2max %: percent of maximal oxygen uptake; VT: tidal volume; VD: physiologic dead space; VA: alveolar volume; VD/VT: ratio of dead space tidal volume; f: breathing frequency; ti: inspiratory time of the breathing cycle; te: expiratory time; ti/ttot: ratio of inspiratory to total time for the breathing cycle;
E: minute volume;
D: dead space ventilation;
A: alveolar ventilation;
E/
O2: ventilator equivalent for oxygen uptake;
E/
CO2: ventilator equivalent for carbon dioxide output; mean Vi: mean inspiratory flow or inspiratory drive; mean
e: mean expiratory flow;
i max: peak inspiratory flow;
e max: peak expiratory flow;
i: volume acceleration at the onset of inspiration;
e: volume acceleration at the onset of expiration.
=0: intrapleural pressure gradient between the two points of zero flow; Cdyn: dynamic lung compliance; RL: total pulmonary resistance; RUA: upper airway resistance; RLA: lower airway resistance; WVIS: viscous work of breathing;
vis: minute work of breathing;
vis/
E: work of breathing per ventilated liter;
vis/
O2: work of breathing per liter of oxygen uptake.
O2: oxygen uptake;
CO2: carbon dioxide output; R: respiratory exchange ratio; venous PO2: venous oxygen partial pressure; venous PCO2: venous carbon dioxide partial pressure; arterial PO2: arterial oxygen partial pressure; arterial PCO2: arterial carbon dioxide partial pressure; HCO3–: bicarbonate content: (A-a)ΔO2: alveolar-arterial oxygen gradient; pHa: arterial pH; PCV: packed cell volume; Hb: blood hemoglobin concentration; arterial SO2: percent saturation of hemoglobin with oxygen; arterial CO2: arterial oxygen content; venous CO2: venous oxygen content; C(a-v)O2: arteriovenous oxygen content gradient; alveolar PO2: alveolar oxygen partial pressure; alveolar PCO2: alveolar carbon dioxide partial pressure; FmeO2: mixed expired oxygen fraction; FmeCO2: mixed expired carbon dioxide fraction; FetO2: end-tidal oxygen fraction; FetCO2: end-tidal carbon dioxide fraction.
: cardiac output; PaPmax: maximal pulmonary artery pressure; PaPmin: minimal pulmonary artery pressure; PaPm: mean pulmonary artery pressure; Pw: pulmonary artery wedge pressure; P (aP-w): pulmonary driving pressure; PVR: pulmonary vascular resistance; tOa: arterial blood temperature;
O2/HR: oxygen pulse;
A/
: global ventilation/perfusion ratio.
Alveolar and dead space ventilation
Distribution of ventilation
Factors that tend to reduce ventilatory asynchrony
Factors that tend to increase ventilatory asynchrony
Pulmonary perfusion
Factors influencing pulmonary vascular resistance
Factors influencing pulmonary perfusion distribution
Bronchial circulation
Lymphatic circulation
Ventilation to perfusion ratio
Pulmonary diffusion
< div class='tao-gold-member'>
Stay updated, free articles. Join our Telegram channel
Full access? Get Clinical Tree
The respiratory system: Anatomy, physiology, and adaptations to exercise and training
Only gold members can continue reading. Log In or Register a > to continue
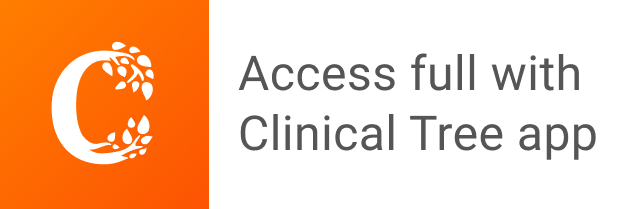