Chapter 15 Skeletal Muscle Function II. SPECIALIZATION OF THE SARCOLEMMA AND SARCOPLASM FOR MUSCULAR CONTRACTION A. Neuromuscular Transmission: Excitation-Conduction B. Coupling Excitation to Contraction III. HETEROGENEITY OF SKELETAL MUSCLE D. Histology and Histochemistry IV. ORIGINS OF FIBER DIVERSITY V. EXERCISE AND ADAPTATIONS TO TRAINING A. Exercise Intensity and Sources of Energy B. Adaptations to Exercise Training VI. DIAGNOSTIC LABORATORY METHODS FOR THE EVALUATION OF NEUROMUSCULAR DISORDERS A. Muscle-Specific Serum Enzyme Determinations Used in the Diagnosis of Neuromuscular Disorders B. Muscle-Specific Serum Proteins and Antibodies VII. MUSCLE BIOPSY AND HISTOCHEMISTRY IN THE DIAGNOSIS OF NEUROMUSCULAR DISORDERS VIII. MOLECULAR DIAGNOSTIC TESTING IX. SELECTED NEUROMUSCULAR DISORDERS OF DOMESTIC ANIMALS B. Cytoskeletal Dystrophin Deficiency and Muscular Dystrophy C. Immune-Mediated Canine Masticatory Muscle Myositis D. Disorders of Glyco(geno)lysis Affecting Skeletal Muscle This chapter outlines skeletal muscle form and function, reviews mechanisms of assessment, and introduces selected neuromuscular diseases that highlight the close relationship between muscle form and function. Skeletal muscle comprises approximately 50% of the body’s mass. It is largely composed of long multinucleated spindle shaped skeletal muscle cells (myofibers) that are highly specialized by virtue of a structured array of muscle-specific contractile proteins and conductile membranes containing ion channels and pumps. Muscles in the body differ in their constituent myofiber populations and vascular and nerve supply, which affects the speed and force developed during muscle contraction as well as the neuromuscular disorders with which they are afflicted. The plasmalemma (sarcolemma) of the skeletal myofiber is an electrically excitable membrane that can activate the contractile machinery in response to signals received from the motor nerve. The properties of excitation and conduction largely result from the presence of membrane-spanning ion conducting pathways and channel gaits that regulate the selective and nonselective conductance of sodium, potassium, calcium, and chloride across the sarcolemma. They activate (open) in response to ligands, transmitters, or changes in voltage and inactivate (close) by intrinsic regulatory processes. Voltage-gated channels contain additional voltage-sensing transmembrane domains and are essential for the generation and modification of action potentials. Ligand-gated ion channels are essential for setting myoplasmic calcium concentrations and establishing signal transduction pathways. Abnormal function of these ion channels produces muscle weakness or altered muscle contractions through altered excitability of the sarcolemma. FIGURE 15-1 Excitation of myofibers to contract involves neuromuscular transmission and the subsequent release of calcium ions into the sarcoplasm. Arrival of an impulse at the axon terminal activates voltage-gated calcium ion channels, resulting in the influx of calcium ions that initiate the calcium-dependent release of the neurotransmitter acetylcholine (ACh) by exocytosis. Liberated ACh diffuses across the synaptic cleft to bind with ACh receptors (two molecules of ACh per receptor) on the postsynaptic sarcolemma. Binding of ACh with AChRs increases the conductance of sodium and potassium ions across the postsynaptic membrane to produce a local end-plate potential at the neuromuscular junction. The end-plate potential generates a muscle action potential that spreads away from the neuromuscular junction in all directions over the surface of the myofiber and into its depths via the transverse (T) tubules. Within the depths of the myofibers, excitation is coupled to contraction through the release of calcium ions from terminal cisternae of the sarcoplasmic reticulum (SR) through calcium release channels of the terminal cisternae. The calcium release channels form small “feet” that extend from the terminal cisternae to the T tubules. The liberated calcium ions bind to the regulatory protein troponin and release the inhibitory action of the regulatory proteins on the contractile events that lead to sliding of the thin (actin) and thick (myosin) filaments. The liberated ACh is subsequently hydrolyzed by AChE (acetylcholinesterase) within the basal lamina of the synaptic cleft. The neuromuscular junction or motor end plate is the synaptic site for chemical transmission of excitation from the presynaptic axon terminal of a motor neuron to the postsynaptic skeletal myofiber (Fig. 15-1). The position of the neuromuscular junction on a muscle fiber can vary among species, among muscles in a species, and among fibers in a given muscle. The axon terminal rests within a primary depression of sarcolemma, the primary cleft, and contains numerous small vesicles that contain acetylcholine (ACh), the neurotransmitter for excitation of skeletal myofibers. Each vesicle contains a quantum of neurotransmitter, consisting of 6000 to 8000 molecules of ACh. Arising from the primary cleft underlying the axon terminal are numerous smaller secondary clefts and complementary folds. The space within the primary and secondary clefts, located between the axon terminal and the postsynaptic sarcolemma, comprises the synaptic cleft. This space is filled with basal lamina containing acetylcholinesterase (AChE). The synaptic basal lamina also plays an important role in the development and regeneration of the neuromuscular junction. The arrival of a nerve action potential at the axon terminal results in activation of voltage-gated calcium ion channels in the presynaptic membrane. The calcium influx initiates a calcium-dependent exocytosis of ACh-containing vesicles from the active zone of the presynaptic membrane. Voltage-gated potassium channels in the presynaptic membrane close the voltage-gated calcium channels and restore resting membrane potential in the axon. The ACh released diffuses across the synaptic cleft to bind with acetylcholine receptors (AChRs), which are concentrated on the crests of the secondary folds of the postsynaptic sarcolemma. The structure of the AChR is similar among animal species. The AChR molecule is an integral transmembrane protein that is formed of five homologous subunits that form a central pore through which ions can flow. It consists of two alpha subunits and single δ, γ, and ε subunits, which possesses a binding site for ACh at the external interfaces of the α/δ and the α/ε subunits. Somewhat deeper within the troughs of the secondary folds are voltage-gated sodium ion channels, which are also present within the sarcolemma throughout nonjunctional regions of the myofiber (Engel, 2004). Excitation of the myofiber is initiated by the reversible binding of ACh with AChRs. The binding of ACh with AChR (two ACh molecules/receptor) results in a local depolarization of the postsynaptic membrane caused by the transient-increased conductance of the AChR cation ion channels to sodium and potassium ions. The amplitude of the end-plate potential (depolarization) is proportional to the number of ACh-AChR complexes formed. At rest, individual quanta of ACh are spontaneously released at a slow rate and cause transient, low-amplitude depolarizations at the end plate. These are referred to as miniature end-plate potentials (MEEPs). The interior of a resting muscle fiber has a resting potential of about –95 mV. The binding of ACh to AChRs is transient, and its effects are abolished by diffusion of ACh away from the receptors and its hydrolysis by AChE. With the arrival of a nerve action potential, approximately 200 quanta of ACh are released, and with the increased number of ACh-AChR combinations, there is a greater conductance of sodium and potassium ions that form a large amplitude depolarization, the end-plate potential (EEP). When the amplitude of the EEP exceeds threshold (approximately –50 mV), a wave of depolarization (muscle action potential, MAP) is generated over the sarcolemma, away from the end plate in all directions. The MAP is propagated by voltage-gated sodium channels over the surface of the myofiber and into its depths via transverse (T) tubules. The T tubules are invaginations of the sarcolemma that tranverse the long axis of the myofiber, and their lumina openly communicate with the extracellular fluid space (Engel, 2004). Excitation-contraction coupling involves the transformation of depolarizing events in the sarcolemma into the initiation of mechanical shortening of the myofibrils within the myofiber by calcium ions released from the terminal cisternae of the sarcoplasmic reticulum (SR). These events occur within the depths of the myofiber at “triads” where the T tubules form junctional complexes with adjacent terminal cisternae of the SR. A triad occurs twice in each sarcomere. The sarcoplasmic reticulum functions in the uptake, storage, and release of calcium ions to regulate the concentration of calcium ions in the aqueous sarcoplasm bathing the myofilaments and other organelles. The concentration of calcium in the SR is aided by the presence of calsequestrin, a calcium-binding protein that is maintained in the lumen of the cisternae by triadin and junctin. At the T-SR junctional complex of triads, the sarcolemma contains voltage-sensitive dihydropyridine receptors (DHPRs) and the terminal cisternae of the SR possess ryanodine-sensitive calcium ion channels (ryanodine receptors) that form “feet” that fill the gap between the terminal cisternae and T tubules. Accessory proteins that regulate ryanodine receptor function include calmodulin and FK-506 binding protein. With depolarization of the sarcolemma within the T tubules, DHPRs interact with ryanodine receptors to mediate the voltage-dependent release of calcium ions from the SR into the sarcoplasm, elevating the calcium ion concentration from 10–7 to 10–5 M. This elevation in calcium ion concentrations initiates contraction through its interaction with the calcium binding proteins such as troponin C and calmodulin, a component of the myosin light chain kinase system (Magleby, 2004). Relaxation is initiated by a reduction in the sarcoplasmic calcium ion concentration through active transport of calcium ions into the lumen of the SR by the SR calcium-ATPase (SERCA). At low calcium concentrations, SERCA activity is inhibited by phospholamban. However, relaxation is promoted by SERCA at higher myoplasmic calcium concentrations generated by stimulation of the ryanodine receptor. Further details concerning the structures and functions involved in neuromuscular transmission and coupling of excitation to contraction are available elsewhere (Engel, 2004; Magleby, 2004; Martonosi and Pikula, 2003; Numa et al., 1990). The ability of skeletal muscle to contract is conferred by the elementary contractile unit, the sarcomere (Fig. 15-2). The sarcomere has three crucial properties: (1) the ability to shorten rapidly and efficiently, (2) the ability to switch on and off in milliseconds, and (3) precision self-assembly and structural regularity. There are three major functional classes of constituent proteins that form the sarcomere: contractile, regulatory, and structural. FIGURE 15-2 Muscular contraction involves the shortening of sarcomeres by sliding of the overlapping arrays of thick (myosin) and thin (actin) myofilaments. The energy for contraction is derived from the hydrolysis of ATP in the presence of an actin-activated ATPase present within the head regions of the myosin thick filament cross-bridges. The ATP is generated by the energy metabolism of the myofiber, principally by anaerobic glycolysis or oxidative phosphorylation. The utilization of ATP may be direct from those sources or indirect from the phosphorylation of ADP from creatine phosphate by creatine kinase (CK). Together, myosin, the principal contractile protein component of thick myofilaments, and actin, the principal component of thin myofilaments, account for more than 70% of myofibrillar protein. Lateral projections of the myosin thick myofilaments (myosin cross-bridges) form reactive sites with actin, which cyclically associate and disassociate during contraction and relaxation. The force-generating step for sliding of the filaments past each other results from changes in the angle of the cross-bridge attachments (Fig. 15-3). a. Thick Myofilaments and Myosin To understand the physicochemical changes that occur at the cross-bridges, the composition and properties of myosin need to be considered. Myosin is an asymmetric protein with both structural and enzymatic properties. It is composed of two identical heavy chains (polypeptide chains with an approximate molecular mass of 200 kDa) and two pairs of light chains (polypeptide chains with molecular masses ranging from 16 to 27 kDa). The two myosin heavy chains are arranged in a double helix to form a long stable tail at one end, and at the other end each heavy chain is folded to form one globular pear-shaped head. The four myosin light chains are contained within the globular heads (two per head) near the junction of the head and neck domains. FIGURE 15-3 Schematic presentation of myosin cross-bridges on thick myofilaments. Portions of the myosin molecules (cross-bridges) project from the thick myofilaments and make contact with the thin myofilaments (a). The light meromyosin (LMM) portion of myosin molecules forms the major structural component (backbone) of the thick myofilament, whereas the heavy meromyosin (HMM) component forms the cross-bridge connections between the thick and thin myofilaments (b). The cross-bridges of myosin are composed of two fractions: (1) the S1 fraction, a globular protein fraction composed of two heads, each possessing binding capacities for ATP and actin and the actin-activated ATPase activity of myosin, and (2) the S2 fraction, a fibrous protein fraction that forms the flexible linkage between the S1 fraction and the LMM portion of myosin (b). The force for sliding of the myofilaments results from a change in the angle of attachment (i.e., a change from 90 to 45 degrees) between the S1 globular head and actin filament (c). The composition of myosin heavy chains within sarcomeres varies among species, among individual muscles, and among individual muscle cells. Mammalian skeletal muscle cells may express six distinct heavy chain genes: perinatal (or neonatal), fast type IIa, fast type IIx (or IId), fast type IIb, and extraocular. The speed of contraction of these myosin heavy chain isoforms increases in the order listed here. Three additional sarcomeric myosin heavy chain genes (super fast, slow A, and slow B) exist, but their expression is unknown, with the exception of expression of superfast myosin in jaw muscles (Sweeney and Houdusse, 2004). A range of myosin light chain isoforms also exist in skeletal muscle that may affect their function. Skeletal muscle possesses both fast skeletal and slow skeletal muscle isoforms of essential light chains as well as regulatory light chains. Biochemically, two classes of light chains can be distinguished: (1) two identical DTNB light chains, which disassociate from their globular heads with the thiol reagent 5,59-dithiobis (2-nitrobenzoic acid) (DTNB), which correspond to regulatory light chains, and (2) two related but different species of 1/alkali light chains (AI and A2), which disassociate at a high pH corresponding to essential light chains (Lowey et al., 1969; Sweeney and Houdusse, 2004; Weeds, 1969). The myosin molecule is composed of head, neck, and tail domains. Proteolytic digestion with trypsin results in the formation of two fragments: (1) heavy meromyosin (HMM), which is composed of the two globular heads of myosin and a short neck domain composed of the initial segment of the fibrous portion; and (2) light meromyosin (LMM), the tail domain composed of the remaining long fibrous portion. The HMM fragments correspond structurally to the cross-bridges, whereas the LMM fragments comprise the bulk of the thick filaments (Fig. 15-3). The actin-binding capacity, ATP-binding capacity, and actin-activated ATPase activity of myosin reside in the globular head. The actin-activated ATPase activity of myosin appears to reside primarily in the heavy chains and is greatly boosted by interaction of the heads with myosin (Sweeney and Houdusse, 2004). b. Thin Myofilaments and Actin The thin filaments are composed of two F-actin strands arranged in a double helical configuration. The F-actin strands are polymers of the globular protein G-actin, and each G-actin monomer possesses a complementary binding site for the myosin globular head. Upon combining with myosin, actin activates the ATPase activity of the myosin globular head (Huxley et al., 1983). Two proteins (tropomyosin and troponin) work in concert with calcium to regulate muscle contraction. Tropomyosin, a fibrous protein, is arranged along the length of the thin filaments, within the grooves of the two F-actin strands. Troponin is a globular protein complex composed of three subunits: TN-I (troponin inhibitory component), TN-T (tropomyosin-binding component), and TN-C (calcium-binding component). The TN-T component attaches the complex to tropomyosin at intervals along the thin myofilaments. With low sarcoplasmic calcium concentrations (10–7 M), tropomyosin molecules block the myosin-binding sites on actin, which prevents the interaction of actin and myosin. At higher concentrations (10–6 M), calcium ions combine with the TN-C component to initiate a conformational change in the TN-I component, which results in the movement of tropomyosin to free the myosin-binding sites on actin. With the myosin-binding site on actin exposed, actin and myosin combine and initiate the cyclical changes associated with that interaction. When calcium ion concentrations are reduced through uptake of calcium by SERCA, the process is reversed and the interaction of actin and myosin is inhibited. The organization of myofilaments within sarcomeres and the organization of myofibrils are supported by a complex cytoskeletal network of intermediate filaments (Wang and Ramirez-Mitchell, 1983). Intermediate filaments, and a number of accessory proteins that form fine filaments, (1) maintain the alignment of myofilaments and sarcomeres, (2) attach and maintain alignment of adjacent myofibrils, (3) attach the sarcomeres of peripheral myofibrils to the sarcolemma, and (4) connect terminal sarcomeres to the sarcolemma at myotendinous junctions. Collectively, the cytoskeletal filaments maintain the structural and functional relationships of the myofilaments and transfer the forces developed by the myofilaments to the sarcolemma. a. Alignment of Myofilaments, Sarcomeres, and Myofibrils Thick myofilaments are attached to Z lines by small filaments composed of the protein titin (Labeit et al., 1997; Maruyama, 1999). The titin filaments arise near the M line within the axes of the thick filaments and span the length of the thick filament as well as the I-band region to attach to the Z line. Within the I-band region, the titin filaments provide an elastic attachment to the Z line, which imparts a passive elasticity to sarcomeres. Myosin-binding proteins attach to the thick filaments and titin and appear to serve a structural role as well as a role in myofibrillogenesis. An additional protein, nebulin, forms small filaments that run the length of thin myofilaments and may regulate the length of thin myofilaments. The M line within the sarcomere stabilizes the thick filament lattice by linking neighboring filaments to each other and has an enzymatic role as well. It is composed of creatine kinase, myomesin, and M protein (Craig and Padron, 2004). At the periphery of myofibrils, adjacent Z lines within the same sarcomere are connected by intermediate filaments of desmin. Also, intermediate filaments of desmin encircle the circumference of Z lines and appear to form linkages with Z lines of adjacent myofibrils to aid in the alignment of sarcomeres in register with adjacent myofibrils. b. Attachment of Myofilaments to the Sarcolemma At the periphery of myofibrils adjacent to the sarcolemma there are riblike attachments (costomeres), which are present on either side of Z lines (Franzini-Armstrong and Horowitz, 2004; Maruyama, 1999). Desmin filaments appear to be anchored to the sarcolemma by a number of adhesion proteins such as vinculin. At myotendinous junctions, the thin myofilaments of the last sarcomere attach to the sarcolemma, which is thrown into numerous villous projections. The thin myofilaments are anchored by the proteins α-actinin and vinculin, among others. Growth in the length of muscle fibers occurs at the myotendinous junctions by the addition of new sarcomeres (Griffin et al., 1971). FIGURE 15-4 Muscular contraction results from the cyclical association and disassociation of actin (A) and myosin (M) in which conformational changes occur in the cross-bridge linkages between the thick and thin myofilaments, associated with the hydrolysis of ATP. Muscular contraction results from the transformation of chemical energy into mechanical energy. The energy for contraction is derived from the hydrolysis of adenosine triphosphate (ATP) into adenosine diphosphate (ADP) and inorganic phosphate (Fig. 15-2) catalyzed by myosin adenosine triphosphatase (ATPase) activity in the myosin head. Chemically, the transformation of energy is associated with the cyclical association and disassociation of the contractile proteins actin and myosin, whereas mechanically the transformation is associated with shortening of sarcomeres, which is achieved by conformational changes of the myosin molecules that result in sliding of the overlapping arrays of thick and thin myofilaments (Fig. 15-4) (Eisenberg et al., 1972; Huxley et al., 1983; Lymn and Taylor, 1971). In the noncontracting state, actin and myosin are combined at the cross-bridges (step 1, Fig. 15-4), and the angle of attachment between the cross-bridge heads and the actin filaments is 45 degrees. Binding of ATP to each globular head (two molecules ATP/myosin molecule) results in a rapid disassociation of actin and myosin (step 2, Fig. 15-4). ATP hydrolysis is rapid when myosin is not associated with actin resulting in the release of phosphate and ADP. The globular head of myosin moves to a new location on the thin filament (step 3, Fig. 15-4), which permits the angle of attachment to become 90 degrees when the globular head recombines with the actin filament (step 4, Fig. 15-4). This recombination step between the globular head and actin is controlled by the regulatory proteins troponin and tropomyosin in response to calcium ion concentrations. The force for contraction is generated by movement of the cross-bridge head to a 45 degree angle of attachment (step 5, Fig. 15-4), and the cycle is completed with the detachment of the hydrolytic products of ATP from the head (step 6, Fig. 15-4). With the formation of ATP through rephosphorylation (step 7, Fig. 15-4), the cycle may be repeated. Measurements indicate that each cycle (stroke) shortens a sarcomere by 12 nm (Barden and Mason, 1978). Rigor mortis, the rigid and stiff condition of skeletal muscles that develops following death, involves cessation of the cross-bridge cycle in the post-force-generating step (step 6, Fig. 15-4). After death, when all ATP stores have been utilized, disassociation of actin and myosin will not occur, and the contraction cycle is terminated with a large number of actin myosin complexes formed with the myosin heads set at 45 degrees. The first indication that different muscles had different physiological prosperities arose from the observation that there was variation in muscle coloration not only among species of animals, but also among individual muscles within the same individual. As a result of these differences in coloration, the terms “red” and “white” were introduced to distinguish between muscles of different gross coloration. Red coloration of muscles was subsequently found to be due to the presence of myoglobin and other cytochromes within the myofibers. Numerous biochemical, histochemical, and physiological studies have since been conducted to detail a variety of differences in both the metabolic and contractile properties of “red” and “white” skeletal muscle. The speed of contraction of red muscles was most often found to be slower than that of white muscles in a variety of animals. In addition, redness of a muscle was associated with the development of tetanus at lower frequencies of stimulation, the development of smaller twitch tensions, and a greater resistance to fatigue. Conversely, white muscles required greater frequencies of stimulation for the development of tetanus, developed larger twitch tensions, and tended to fatigue quickly. From this data, the terminology of slow-contracting or slow-twitch and fast-contracting or fast-twitch muscles evolved. Moreover, because speed of contraction was closely associated with gross muscle coloration, the terms “red” and “white” came to be used interchangeably with “slow” and “fast,” respectively. However, there are numerous exceptions to this association of gross coloration with physiological properties of contraction. Therefore, direct associations must not be assumed. The morphological and functional unit of skeletal muscles is the motor unit (Fig. 15-5). The motor unit is composed of (1) the motor neuron, consisting of its cell body located within the central nervous system (selected cranial nerve nuclei or the ventral horn of the spinal cord), and its axon, which extends along the ventral root and peripheral nerve; (2) the neuromuscular junctions; and (3) the myofibers innervated by the motor neuron. FIGURE 15-5 Schematic representation of the homogeneity of skeletal muscle motor units. Motor units have a homogeneous myofiber-type composition whereby slow-twitch motor units are composed of only type 1 myofibers (light staining for myosin ATPase), whereas fast-twitch units are composed of only type 2a (fast-twitch, fatigue resistant) myofibers, or only type 2b (fast-twitch, fatigable) myofibers (dark staining for myosin ATPase). Motor neurons may differ based on their rates of discharge: (1) phasic motor neurons with a fast discharge rate and (2) tonic motor neurons with a slow discharge rate. In addition, the phasic motor neurons are characterized by shorter after hyperpolarization potentials, faster conduction velocities, and larger axons than the tonic motor neurons. Investigations of these parameters in motor neurons of slow-contracting and fast-contracting muscles indicate that tonic motor neurons, which discharge at rates of 10 to 20 per second, innervate slow-contracting muscles, and phasic motor neurons, which discharge at rates of 30 to 60 per second, innervate fast-contracting muscle. Thus, there are at least two types of motor units, which differ in their physiological properties and type of motor neuron innervation (Eccles et al., 1958). Physiological measurements performed on isolated motor units in the cat have revealed two types of fast-twitch motor units and one type of slow-twitch unit (Burke, 1975). Some fast-twitch motor units are resistant to fatigue and designated FR units (i.e., fast-twitch, resistant to fatigue); others fatigue rapidly and are designated FF units (i.e., fast-twitch, fatigable). All of the slow-twitch units are resistant to fatigue and are therefore designated as S units (i.e., slow-twitch). The average number of muscle fibers per motor unit in cats ranges from 550 to 650. Subsequent immunohistochemical studies (discussed later) reveal that slow-twitch motor units contain type I myosin isoforms, and most FR motor units contain type IIa or IIa/x myosin isoforms and most FF motor units contain type IIx (mammals) or IIb (rodent) myosin isoforms. FIGURE 15-6 Serial sections of cat medial gastrocnemius muscle incubated for the histochemical demonstration of (a) myofibrillar ATPase, incubated at pH 9.4; (b) myofibrillar ATPase, preincubated at pH 4.5; (c) NADH-tetrazolium reductase. Type 1 myofibers comprise slow-twitch motor units that are resistant to fatigue. Type IIA myofibers comprise fast-twitch motor units that are resistant to fatigue, whereas type IIB myofibers comprise fast-twitch motor units that fatigue rapidly. Dark NADH staining indicates high mitochondrial content; and (d) myofibrillar ATPase, preincubated at pH 4.2. a. Histoenzymic Properties Associated with Myosin-ATPase Myofibers have been differentiated histochemically into type I and type II myofibers based on their staining reaction for myosin ATPase (Fig. 15-6) (Dubowitz and Brooke, 1973). Furthermore, type II myofibers, classified by the myosin ATPase staining reaction, may be further subdivided into type IIA, type IIB, and type IIC myofibers based on the lability of their ATPase activity following preincubation in acid and alkaline media (Fig. 15-6) (Brooke and Kaiser, 1970). If the actin-activated myosin ATPase activity is rate limiting in the speed of contraction, it follows that the histochemical method for myosin ATPase would be a specific method for the differentiation of myofiber types based on their speed of contraction. This classification scheme is generally applicable to all mammalian species except for canine muscles, which do not contain classical type IIB myofibers (Braund et al., 1978; Orvis and Cardinet, 1981). b. Immunocytochemical Identification of Myosin Isoforms More recently, immunohistochemical differentiation of fiber types based on antibodies directed against myosin heavy chain isoforms have been used to identify contractile muscle fiber types (Gorza, 1990). To henceforth distinguish between the two methods for fiber-type identification roman numerals will be used for histochemical fiber types and Arabic numbers will be used for myosin isoforms. Immunohistochemical staining for myosin isoforms reveals that the true number of distinctly identified muscle fiber types supersedes the number recognized by histochemistry alone. Myosin isoforms include neonatal and slow-twitch myosin isoforms as well as five distinct fast-twitch isoforms (Rubenstein and Kelly, 2004). Type 2a, type 2b, and type 2x are the most widely expressed skeletal muscle isoforms in the body with 2 m fibers found in the jaw muscles of carnivores and 2eom in extraocular muscles. Unfortunately, type IIB fibers distinguished histochemically by myosin ATPase activity do not correspond to type 2b fibers distinguished by immunhistochemical staining for myosin heavy chains. Rather type IIB fibers correspond more closely with type 2x fibers (also called 2d) found in many mammalian species whereas 2b fibers correspond to myosin found in rapidly contracting muscle fibers found in rodents and camelids (Gorza, 1990). Moreover patterns of contractile protein isoforms and enzyme activities recognized in developing or pathological muscles do not correlate with standard histochemically derived fiber types (Linnane et al., 1999). Hence, though useful as a screening tool for pathologists and physiologists, histochemical techniques have limitations. Most limb muscles are “mixed” and contain variable proportions of type 1, type 2a, and type 2x myofibers. Intermediate fiber types called type IIC by myosin ATPase histochemistry or neonatal by immunohistochemistry are normally rare in mature muscle. These fibers presumably represent fibers capable of transitioning between type 1 and type 2 myofibers (Brooke and Kaiser, 1970). c. Histoenzymic Properties Associated with Aerobic and Anaerobic Energy Metabolism Histochemical studies show that type 1 myofibers have higher activities of oxidative enzymes such as succinate dehydrogenase (SDH), reduced nicotinamide adenine dinucleotide tetrazolium reductase (NADH-TR) (Fig. 15-6), and reduced nicotinamide adenine dinucleotide phosphate-tetrazolium reductase (NADPH-TR) than type 2 fibers (Dubowitz and Brooke, 1973). In conjunction with electron microscopic observations, the activities of these enzymes have been localized to mitochondria, which are present in abundance in type I fibers. Associated with the large mitochondrial volume of type 1 myofibers are lipid inclusions. Type II fibers in general stain darkly with glyco(geno)lytic stains such as phosphorylase or phosphofructokinase activity. Because various intermediate histochemical reactions of myofibers also exist, a classification system that describes type 1, type 2 oxidative, and type 2 glycolytic is often used. d. Relationships with Functional Properties Each motor unit is homogeneous with respect to its myofiber-type composition. Motor units with slow-twitch fibers are fatigue resistant and corresponded to type 1 fibers in histochemical stains. Two types of fast-contracting motor units exist; fast-twitch fatigue resistant, corresponding to type 2a fibers, and fast-twitch rapidly fatigable corresponding to type 2x fibers identified histochemically (Burke, 1975). FIGURE 15-7 Schematic representation of some differences in energy-yielding metabolic pathways of slow-twitch and fast-twitch muscles. In slow-twitch muscles, energy for contraction is derived primarily by oxidative phosphorylation resulting from the oxidation of fatty acids, carbohydrates, and perhaps amino acids via the tricarboxylic acid cycle (TCA). Fast-twitch muscles derive their energy primarily via anaerobic glycogenolysis and glycolysis through the degradation of glycogen and glucose to lactate. Aerobic glycolysis via the HMP (hexose monophosphate) shunt is a minor pathway in both types of muscle. The physiological and histochemical properties and classifications of myofibers are summarized in (Table 15-1) and illustrated in Figure 15-6 and Figure 15-7. As previously discussed, the actin-activated myosin ATPase activity catalyzes the cyclical physiochemical interactions of actin and myosin during contraction (Fig. 15-4). Furthermore, the intrinsic speed of contraction (sarcomere shortening) has been demonstrated to be proportional to the activity of actin-activated myosin-ATPase, and differences exist between the myosin-ATPase activities of type I and type II muscle fibers. From those observations it is postulated that the rate-limiting step of sarcomere shortening during contraction is the hydrolysis of ATP. The ATPase activity of type I fibers is lower than in type II fibers, and their pH dependency and liability in acid and alkaline conditions also differ (Barany, 1967; Seidel, 1967). Quantitative differences in enzyme activities and various substrate concentrations have been reported between slow-twitch type 1 and fast-twitch muscle fibers type 2 as well as between fibers expressing myosin isoforms type 2a and 2x. Those biochemical differences between fiber types reflect differences in their principal metabolic pathways active in the generation of energy (ATP) for muscular contraction (Fig. 15-7). The ATP required for contraction is not stored in significant quantities. Therefore, ATP must be readily produced through the metabolism of fats, carbohydrates, and creatine phosphate stores to support the energy requirements for contraction. Aerobically, ATP is produced in muscle mitochondria by oxidative phosphorylation coupled to electron transport. Anaerobically, ATP is produced in the aqueous sarcoplasm through substrate phosphorylation of ADP by (1) creatine kinase, utilizing creatine phosphate stores; (2) glyco- (geno)lysis, utilizing muscle glycogen stores, and (3) myokinase kinase, utilizing ADP produced by the ATP hydrolysis. a. Aerobic and Anaerobic Energy Metabolism In general, fast-twitch muscle fibers in the untrained state are biochemically suited to derive energy for contraction by anaerobic glyco(geno)lysis. Fast-twitch fibers, particularly type IIx, tend to have higher concentrations of glycogen and creatine phosphate as well as higher activities of enzymes associated with glycogenolysis and glycolysis (Table 15-1). Slow-twitch type I fibers, on the other hand, generally have higher concentrations of triglycerides and myoglobin and are better suited to derive their energy by oxidative phosphorylation via the electron transport system following the oxidation of fatty acids and glucose via the Krebs cycle (Table 15-1). Type IIa fibers are intermediate in their glycolytic and oxidative capacity between type IIx and type I fibers (Adhihetty et al., 2003; Rubenstein and Kelly, 2004). Triglycerides and glycogen serve as primary substrates for muscle metabolism. In general, the rate of glycogen utilization is greatest with high-intensity anaerobic exercise, whereas low-intensity submaximal exercise results in a lower rate of glycogen utilization and reliance on oxidation of fatty acids as fuel (Kiens, 2006). Under conditions of restricted energy intake or prolonged exercise, amino acids may also serve as energy substrates within skeletal muscle (Rennie et al., 2006). b. Purine Nucleotide Cycle With strenuous exercise when the regeneration of ATP fails to meet energy demands, the myokinase reaction can be used to generate ATP from accumulated ADP. The accumulation of the additional product of this reaction, AMP, stimulates the purine nucleotide cycle, which removes AMP thereby preventing product inhibition of the myokinase reaction. The deamination of AMP by AMP deaminase in the purine nucleotide cycle results in the production of ammonia and inosine monophosphate (IMP) (Lowenstein, 1972). The cycle further involves the regeneration of AMP through deamination of aspartate and the hydrolysis of guanosine triphosphate to form adenylosuccinate, and the cleavage of adenylosuccinate to form fumarate and AMP. The activity of AMP deaminase is greater in type II compared to type I muscle fibers. The purine nucleotide cycle regulates energy requirements for muscular contraction through (1) maintenance of a high ATP:ADP ratio by regulating the relative AMP, ADP, and ATP levels; (2) regulation of phosphofructokinase (PFK) activity through activation of this enzyme by ammonia; (3) regulation of phosphorylase activity through activation by IMP; (4) replenishment of citric acid cycle intermediates by the production of fumarate; and (5) deamination of amino acids for oxidative metabolism through the formation of aspartate (Tullson and Terjung, 1991). From the foregoing, it is evident that the deamination of AMP can promote ATP synthesis by (1) stimulating anaerobic glyco(geno)lysis through the activation of phosphorylase b by IMP, and the activation of PFK by ammonia, and (2) supporting oxidative metabolism through the production of intermediates into the TCA cycle. The degradation of adenine nucleotides in equine muscle appears to occur mainly through deamination of AMP. Reported AMP deaminase activities are greatest for equine middle gluteal muscles, which were approximately double reported values for muscles of the rat and rabbit (Cutmore et al., 1986). c. AMP Kinase Another emerging key sensor and regulator of energy metabolism in skeletal muscle is AMP-activated protein kinase (AMPK), which monitors astonishingly small shifts in the cellular AMP:ATP ratio (Hardie, 2004). The activation of AMPK—either by allosteric interactions of AMP, phosphorylation by AMPK kinase, or both—triggers a shift from energy-consuming pathways, such as glycogen, fatty acid, and cholesterol synthesis, to ATP-generating pathways via the phosphorylation of key regulatory enzymes (Carling, 2004). AMPK activity may be necessary for contraction-stimulated glucose transport. AMPK has also been implicated in regulating gene transcription and, therefore, may function in some of the cellular adaptations to training. Most muscles in domestic animals contain a mixture of muscle fiber types (Fig. 15-6). The muscle fiber composition, the percentage of type 1, 2a, and 2x fibers, and muscle fiber cross-sectional areas vary greatly among species, muscle groups, individuals, and breeds. When comparing the fiber-type composition of different individuals, a standardized site must be used as fiber-type proportions vary along the length and depth of a muscle. Locomotor muscles in most domestic animals have a combination of type 1, 2a, and 2x fibers (or I, IIA, and IIB depending on the technique used for fiber typing). Locomotor muscles in dogs contain type I and type IIA fibers and no type 2B fibers using ATPase stains for fiber typing; however, histochemical type IIA fiber types appear to correspond to both type 2a and hybrid type 2a/x MHC isoforms (Strbenc et al., 2004). Camelid muscles contain an unusual mixture of 1, 2a, 2b, and 2x fibers (Graziotti et al., 2001). Horses have a high proportion of type 2a and 2x fibers relative to type 1 fibers in their locomotor muscles. Breed differences have been extensively studied in horses (Snow and Valberg, 1994). In general, quarter horses and Thoroughbreds have the highest percentage of fast-twitch muscle fibers, 80% to 90%; standardbreds have an intermediate number, 75%; and donkeys have the lowest percentage of fast-twitch fibers in locomotor muscles (Snow and Valberg, 1994). Fiber-type composition is not constant, as growth and training can alter the fiber-type composition and fiber size in the same muscle over time. With growth and training, there is a change in the length and breadth of a fiber as well as a change in the proportion of fiber types rather than an increase in the number of muscle fibers. Growth and training at speed results in an increase in the proportion of type IIA (2a) fibers and a concomitant decrease in type IIB (2x) fibers (Eto et al., 2003, 2004). When a muscle contracts during exercise, it does so in response to a predetermined recruitment of particular muscle fibers. This orderly recruitment of muscle fibers leads to smooth, coordinated movement. As exercise begins, a select number of motor units are recruited to provide the power to advance the limb. Motor units are recruited with respect to their contractile speed and oxidative capacities (Burke, 1975; Valberg, 1986). At slow exercise intensities, type I and a small number of type II fatigue-resistant muscle fibers are stimulated. The force produced by any muscle is proportional to the cross-sectional area that is active. As the speed or duration of exercise increases, more muscle fibers are recruited, and this occurs in the order of their contractile speed from type I to type IIA and type IIB (Lindholm et al., 1974; Valberg, 1986). With moderate intensity, type I and type IIA myofibers are preferentially recruited, whereas moderate intensity of long duration or maximal exercise intensity is required for recruitment of type IIB myofibers. More recent studies suggest that muscle fiber recruitment may be regulated by the central nervous system, with the subconscious brain producing an anticipated regulated response governed by peripheral feedback mechanisms and predetermined patterns of recruitment acquired from training and modulated by conscious motivation (Noakes et al., 2004). The origins of muscle fiber-type composition appear to lie in lineage directives that developing embryonic myoblasts obtain from their progenitors, which limit to some extent the plasticity of the adult myofibers (Rubenstein and Kelly, 2004). Specification to become slow- or fast-twitch fibers appears to exist already in the myoblast stage and manifests when myotubes express one or the other MHC isoform. Subtypes of fast-twitch fibers become established following the commitment to the fast-twitch phenotype and occur in concert with the development of thyroid function (Russell et al., 1988). In the embryo, the primordial myoblasts migrate to their position in the limb of the embryo where their fiber type is further influenced by temporal and positional factors, synaptogenesis, imposed neuronal activity, and activation of specific signal transduction pathways. At least two signal transduction pathways may contribute to fiber-specific synthesis of slow myosin. These include calcineurin, a calcium-regulated serine/threonine phosphatase, and Ras (Rubenstein and Kelly, 2004). A mosaic of fiber types subsequently forms with fiber-type predominance programmed in certain muscles or portions of muscles. In mature muscle, the nerve has important trophic influences on the innervated muscle, which regulate its structural and metabolic properties. Motor units contain fibers of the same type. When motor neurons that normally innervate slow muscles are cross-innervated to supply muscles that are normally fast, and motor neurons that normally innervate fast muscles come to innervate muscles that are normally slow, a reversal of contractile properties occurs—that is, fast-twitch muscles become slow, and slow-twitch muscles become fast. Accompanying this is a corresponding change in the enzyme histochemical profiles of the myofibers. Therefore, the motor neuron influences (1) the type of energy metabolism employed by a myofiber and all the structural changes in fiber organelles that this implies and (2) the myofiber’s physiological properties of contraction. More recent cross-innervation studies, however, demonstrate that the embryogenic determination of muscle fiber types provides an inherent bias toward an original phenotype. Transplantation of myoblasts from cat jaw muscles (type 2 m fibers) into the fast-twitch extensor digitorum limb muscle results in expression of their 2 m myosin isoform even when enervated by the extensor digitorum nerve (Hoh and Hughes, 1988). Conversely, when jaw muscle myoblasts are transplanted into the slow-twitch soleus muscle, the soleus nerve can override type 2 m expression and switch fibers to myosin heavy chain type 1 expression. Taken together, these studies suggest that muscle fiber type is determined by competitive interactions between endogenous programming and exogenous cues from motor neurons that require continual reinforcement (Rubenstein and Kelly, 2004).
I. INTRODUCTION
II. SPECIALIZATION OF THE SARCOLEMMA AND SARCOPLASM FOR MUSCULAR CONTRACTION
A. Neuromuscular Transmission: Excitation-Conduction
B. Coupling Excitation to Contraction
C. Muscular Contraction
1. Myofilaments and Contractile Proteins
2. Regulatory Proteins
3. Structural Proteins
D. Muscular Energetics
III. HETEROGENEITY OF SKELETAL MUSCLE
A. Gross Muscle Coloration
B. Physiological Properties
C. Motor Units
D. Histology and Histochemistry
1. Histochemical Properties of Myofibers
E. Quantitative Biochemistry
1. Metabolic Pathway for Utilization of Energy (ATP) for Contraction
2. Metabolic Pathways That Generate Energy (ATP) for Contraction
F. Muscle Fiber Composition
G. Muscle Fiber Recruitment
IV. ORIGINS OF FIBER DIVERSITY
V. EXERCISE AND ADAPTATIONS TO TRAINING
A. Exercise Intensity and Sources of Energy
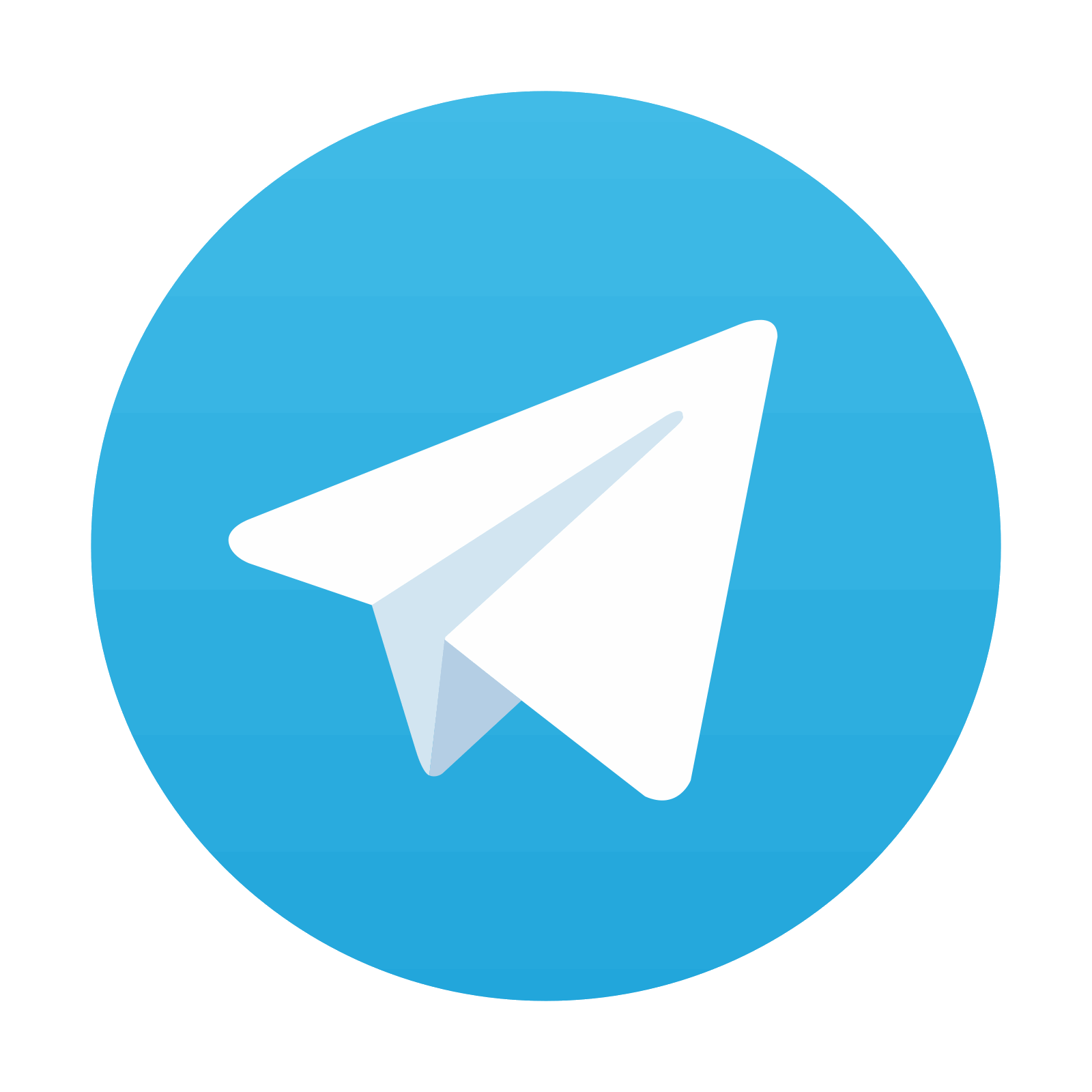
Stay updated, free articles. Join our Telegram channel
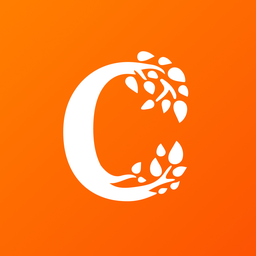
Full access? Get Clinical Tree
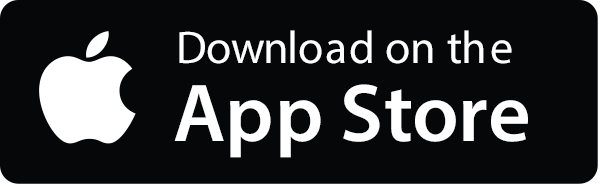
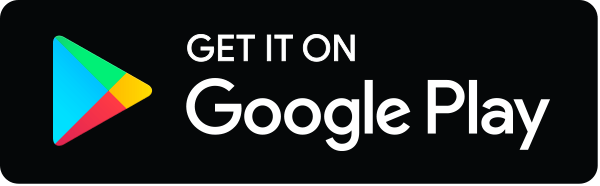