14 Lysa P. Posner Sedatives and tranquilizers are commonly used in veterinary medicine to produce calmness, for chemical restraint, and as an adjunct with general anesthesia. Drugs that produce sedation often have different mechanisms of actions, pharmacodynamic effects, analgesic properties, reversibility, and physiological effects. It is therefore important to understand the different classes of sedative available to be able to predict wanted effects and potential side effects. Phenothiazines were originally developed and used as antipsychotic drugs for the treatment of schizophrenia in people. However, they have been used in veterinary medicine as tranquilizers since the 1950s. The term neuroleptic (antipsychotic) was first used to describe the effects of phenothiazines on the central nervous system, but the more common term, major tranquilizer, is still used. The most commonly used phenothiazine in veterinary medicine is acepromazine (ACP). It is incorrectly referred to as “acetylpromazine” in some publications. It is unique because it is not used in human medicine, but has been widely used in veterinary medicine for decades. Phenothiazine derivative, major tranquilizer, and antiemetic. Phenothiazine drugs produce sedation by inhibiting postsynaptic central dopaminergic receptors (D2). Dopamine receptors are included in the family of G-protein-coupled receptors and act as a first messenger by interacting with the receptor proteins of the postsynaptic membrane. This interaction results in transduction of the signal by a guanine nucleotide-binding regulatory protein (G protein) to an appropriate intracellular effector system, or second messenger. Therefore antagonism of the D2 receptor decreases neurotransmission resulting on behavioral changes (calming and sedation). Phenothiazines also have antiemetic effects, which are from D2 antagonism at the chemoreceptor trigger zone (Peroutka and Snyder, 1982). Peripherally, and as a side effect, the phenothiazines block the binding of norepinephrine at α-adrenergic receptors (α1 antagonism). Blockade of these receptors results in peripheral vasodilation. Phenothiazines are commonly administered for routine sedation in a variety of species. They are also used in the perianesthetic period to reduce anxiety, reduce induction and maintenance drugs doses, decrease vomiting in susceptible animals, improve the quality of induction and recovery, and to contribute to more balanced anesthesia. Phenothiazines are commonly administered with opioids (e.g., morphine) to produce neurolept analgesia; the combination produces synergic effects of each class (i.e., greater sedation and greater analgesia). Phenothiazines can also prevent nausea and vomiting, particularly the vomiting associated with opioid administration (Valverde et al., 2004). All phenothiazines produce a sedation by depressing dopaminergic neurotransmission but vary in potency and duration of action. Phenothiazines decrease spontaneous motor activity in animals, but should not affect coordination. Although sedation is generally reliable, arousal is easily accomplished, particularly in excited or aggressive animals. Extrapyramidal symptoms (rigidity, tremor, akinesia) can be an adverse effect of phenothiazines, particularly at high doses. Phenothiazines as a class (including acepromazine) have been considered contraindicated for patients with seizure histories, due to a series of experiments in rabbits that showed alteration of the seizure threshold with a proconvulsant drug in patients administered chlorpromazine (Fabisch, 1957). However, there is little scientific evidence that acepromazine induces spontaneous seizures in veterinary patients and recent studies have disputed this conclusion and have even suggested that acepromazine may be anticonvulsant (Tobias et al., 2006; McConnell et al., 2007). In addition to blockade of the central effect of dopamine, phenothiazines block peripheral α1-adrenergic receptors in the vasculature, which results in peripheral vasodilation, and can produces systemic hypotension and a decrease in cardiac output (Stepien et al., 1995). Healthy animals show mild changes to arterial blood pressure; however, the hypotensive effects may be exaggerated in patients that are anesthetized, debilitated, or hypovolemic. A mild reflex tachycardia may be seen in response to hypotension (Turner et al., 1974). Vasoconstrictors, such as phenylephrine, can be used to attenuate the hypotensive effects of phenothiazines (Ludders et al., 1983). The vasodilatory effects generally last longer than the sedation. Phenothiazines should be used cautiously with locoregional (epidural and intrathecal) anesthetic procedures because they might potentiate the arterial hypotensive effects of local anesthetics. Acepromazine decreases ventricular arrhythmias by raising the arrhythmogenic dose of epinephrine (Muir et al., 1975), even at low doses (Dyson and Pettifer, 1997). Clinical doses of phenothiazines ordinarily have mild effects upon respiratory activity. Respiratory rate is often depressed, but minute volume remains normal (i.e., no change in CO2). However, respiratory depression may be seen or exaggerated when phenothiazines are administered with other CNS or respiratory depressants (e.g., opioids, isoflurane) or when used at high doses. Acepromazine attenuates the shunt of ventilation perfusion mismatches seen horses sedated or anesthetized with α2-agonists and or dissociative anesthetics (Marntell et al., 2005) (see Section Species Differences, Horse). Phenothiazines provide good muscle relaxation and are often used in conjunction with anesthetics that do not provide muscle relaxation or that result in muscle rigidity (e.g., ketamine). The phenothiazine, acepromazine, has been shown to decrease the incidence of malignant hyperthermia in pigs (see Section Species Differences). Phenothiazines in general have a large volume of distribution (Vd) and are highly protein bound. In the horse, acepromazine Vd = 6.6 l/kg and is >99% protein bound (Ballard et al., 1982). Time to clinical effect is generally ∼10–15 minutes after IV administration and ∼30 minutes after IM administration. Acepromazine is long acting in most species; elimination half-life is 3.1 hours in the horse and 7.1 hours in the dog (Ballard et al., 1982; Hashem et al., 1992). Acepromazine undergoes extensive hepatic metabolism and metabolites are excreted in the urine (Dewey et al., 1981). The metabolites of acepromazine have not been evaluated extensively in most species, but horses and humans have differing metabolites and in differing concentrations (Dewey et al., 1981; Elliott and Hale, 1999). Bioavailability in dogs after oral administration is ∼20% (Hashem et al., 1992), which must be accounted for if tablets are administered instead of injection. There is no available antagonist to reverse the effects of phenothiazines. Acepromazine use is associated with decreased perianesthetic morbidity and mortality in horses (Johnston et al., 2002). Patients administered phenothiazines cannot thermoregulate appropriately. Phenothiazines alter thermoregulation by decreased catecholamine binding in the hypothalamus (where thermoregulation is controlled centrally) as well as by altering vasomotor tone in the peripheral vessels that participate in heat retention and elimination. Following administration, patients should be protected from extreme temperature fluctuations (e.g., housed in the sun or in the cold). The use of acepromazine in patients with coagulopathies is controversial. Acepromazine has been shown to decrease platelet aggregation, which alters coagulation times (Barr et al., 1992). Conversely, administration of acepromazine did not change platelet function via thromboelastography (Conner et al., 2012). Clinically, acepromazine has rarely been implicated in abnormal bleeding. Recommendations for acepromazine use are clinician dependant, although careful consideration in patients with abnormal number or function of platelet, or overt coagulopathy would be prudent. Phenothiazines markedly reduce the hematocrit of animals (Parry and Anderson, 1983; Robertson et al., 2001; Ballard et al., 1982). The decrease in packed-cell volume (PCV) by phenothiazines is likely due to splenic sequestration of red blood cells as well as translocation of fluids in response to hypotension. Blood samples drawn from phenothiazine-treated animals for diagnostic purposes should be interpreted accordingly. Phenothiazines are antiemetic and have been shown to decrease vomiting in patients when administered with drugs that cause vomiting (e.g., opioids) (Valverde et al., 2004). The antiemetic effects are presumably from the blockade of dopamine receptors in the chemoreceptor trigger zone within the medulla (Peroutka and Snyder, 1982). Phenothiazine derivatives can produce phototoxicity (Elisei et al., 2002), which has been induced in laboratory animals following administration of chlorpromazine in the presence of black-light irradiation (Akin et al., 1979). Although phototoxic reactions in animals do not appear to be an important clinical problem, those with scant hair and white hair probably should not receive excessive exposure to sunlight during treatment with phenothiazines. Phenothiazine drugs have antihistaminic (H1) properties (Lichtenstein and Gillespie, 1975). These effects are clinically relevant and these drugs should not be used for skin testing where histamine-caused wheal formation is assessed (Moriello and Eicker, 1991). Although potentially useful for preventing histamine release, the hypotension associated with phenothiazine drugs would preclude their use to treat abnormal histamine release (e.g., mast cell degranulation). Phenothiazine derivatives provide little or no analgesic activity (Barnhart et al., 2000). Tranquilization with these drugs must be supplemented with analgesics and/or general anesthetics to block nociceptive responses during painful procedures. Many phenothiazines are used to treat psychiatric problems in people and animals. The following three phenothiazines are currently used in veterinary medicine to produce sedation or as an adjunct to anesthesia. Acepromazine is the most commonly used phenothiazine in veterinary medicine. It is routinely used for sedation of many veterinary species, although it only approved for use in dogs, cats, and horses. It is approved for administration IV, IM, or SQ. Acetylpromazine, Vetranquil, ACE, ACP. 1-[10-[3-(dimethylamino) propyl]-10H-phenothiazin-2-yl ethanone. C19-H22-N2-O-S (Figure 14.1). Figure 14.1 Structure of phenothiazines. 442.5334. Acepromazine is available in yellow aqueous solution at 10 mg/ml and in 5, 10, and 25-mg tablets. The solution should be protected from light. see Box 14.1. Promazine is phenothiazine similar to acepromazine. It is rarely used in the parenteral form, but is more popular as a granular oral medication that is mixed with feed for use in horses. Promazine granules, promazine. 10-(3-dimethylaminopropyl)phenothiazine (Figure 14.1) C17-H20-N2-S.Cl-H. 442.5334. Promazine granules, 27.5 mg/gram for mixing with feed For horses, mix 1–2 mg/kg of granules with feed. For cattle, mix 1.5–2.75 mg/kg with feed. Chlorpromazine is primarily used as an antiemetic in dogs and cats, and less commonly used as a preanesthetic sedative. It is generally contraindicated for use with horses, due to a high incidence of ataxia and altered mentation. 10-(3-Dimethylaminopropyl)-2-chlo- rophenothiazine monohydrochloride. C17-H19-Cl-N2-S.Cl-H (Figure 14.1). For the dog and cat, administer 0.55–2.0 mg/kg IV, IM to produce sedation. The use of acepromazine in the perianesthetic period is associated with decreased risk of anesthetic morbidity and mortality in horses (Johnston et al., 2002). Acepromazine have been associated with prolonged paraphimosis (penile prolapse) in the horse. Routine sedative doses are generally associated with paraphimosis, which generally resolves within 30 minutes (Ballard et al., 1982). However, in some horses prolapse can remain for greater than 100 minutes, causing swelling (likely in association with vasodilation), trauma, and failure for the penis to retract normally, which creates a management issue and potential for loss of breeding function. In the USA, acepromazine is used with caution in breeding horses, but that concern may be overrated (Driessen et al., 2011). In many parts of the world (e.g., United Kingdom), acepromazine is routinely used for equine anesthesia and has even been associated with better anesthetic outcomes in horses (Johnston et al., 2002). Anecdotal reports suggest pathological paraphimosis does not occur when the total dose does not exceed 10 mg/horse, which was supported retrospectively (Driessen et al., 2011). Acepromazine attenuates the shunt of ventilation–perfusion mismatches seen horses sedated or anesthetized with α2-agonists and/or dissociative anesthetics (Marntell et al., 2005). The pulmonary effects are likely due to the combination of improving cardiac output and permitting normal hypoxic pulmonary vasoconstriction (Marntell et al., 2005). Acepromazine produces α1-adrenergic blockade, which balances the vasoconstriction of α2-agonist sedative drugs. Vasoconstriction and the reflex bradycardia associated with α2-agonists are major causes of decreased cardiac output. Additionally, the rise in pulmonary arterial pressure (PAP) associated with α2-agonists and dissociative anesthetics likely interfere with hypoxic pulmonary vasoconstriction. Hypoxic pulmonary vasoconstriction is the normal mechanism to limit ventilation and perfusion mismatches. Chlorpromazine is not recommended in horses due to extreme ataxia and altered mentation. Some Boxer dogs have an exaggerated response to the phenothiazine, acepromazine (Brock, 1994). In those dogs, sedation and hypotension are greater than expected and there have been anecdotal reports of syncopal episodes, presumably from hypotension. Aggressive symptomatic treatment of hypotension has resulted in full recoveries. Therefore acepromazine might be avoided all together in Boxer dogs or used at much reduced doses with monitoring for any untoward effects. Dogs with high vagal tone (Bulldogs, Boxers) have anecdotally been reported to have greater morbidity and mortality when administered acepromazine, presumably caused by the adrenergic blocking effects of the drug. Similar recommendations for avoidance or reduced dosages have been made. Acepromazine may prevent the occurrence of halothane-induced malignant hyperthermia in susceptible pigs (McGrath et al., 1981). All phenothiazines should be used cautiously with any drugs that also produce vasodilation or hypotension. All phenothiazines when administered with other CNS depressants can produce exaggerated CNS depression. Doses of one or both drugs might need to be reduced. The phenothiazines have very high therapeutic indexes, particularly when used in conscious (nonanesthetized) animals. (Stoelting, 1999). Dogs that received more than 100 times the recommended oral dose had no fatalities. This safety margin is likely diminished in anesthetized patients where many protective reflexes are attenuated. Phenothiazines should be avoided in patients that are dehydrated, hypovolemic, bleeding, or in shock because of the drugs effect on vessel tone (vasodilation). Phenothiazines should be used cautiously in patients with coagulopathies or thrombocytopenia due to their effect on platelet aggregation (see Section Miscellaneous). Phenothiazines should be used cautiously in Boxer dogs, brachiocephalic dogs, breeding stallions, or debilitated animals. Acepromazine is a nonscheduled drug. No listed withdrawal times for the dog, cat, or horse; however, phenothiazines should not to be used in horses intended for human consumption. Acepromazine is approved by the FDA for use in dogs, cats and horses. Promazine is approved by the FDA for use in dogs, cats, and horses. There are no veterinary approved products. It is supplied as 25 mg/ml injection for human use (Thorazine, and generic brands), and is used off label in veterinary patients. It is also supplied in 10, 25, 50, 100, and 200-mg tablets for people. Butyrophenone drugs (haloperidol and droperidol) were first introduced into human medicine in the late 1950s as antipsychotics. Haloperidol is still used as an antipsychotic and droperidol is primarily used to prevent postoperative nausea and vomiting. Droperidol was used in veterinary medicine primarily as a sedative, but is no longer marketed due to adverse behavioral effects. There was previously a formulation called “Innovar-Vet”, which was a combination of droperidol and fentanyl citrate for injection, but this product is no longer marketed. More information regarding droperidol may be found in previous editions of this text. Butyrophenones are less commonly used in veterinary medicine; however, azaperone is still used for sedation in veterinary medicine in swine and zoo/wildlife medicine. Butyrophenones are neuroleptic sedatives, and still one of the major classes of antipsychotic drugs in use in human medicine. The CNS effects of butyrophenones are primarily due to the antagonism of D2 receptors in mesolimbic–mesocortical pathways in the brain, but there is also antagonism at D1, 5-HT, α1, and histamine receptors (Potter and Hollister, 2001). Some of the adverse signs associated with butyrophenones, such as extrapyramidal signs, tardive dyskinesis, muscle tremors, and restlessness, are associated with dopaminergic antagonism of the nigrostriatal pathways (Potter and Hollister, 2001). Although butyrophenones produce α1-adrenergic receptor antagonism (vasodilation), there is less affinity for these receptors, which explains why butyrophenones do not cause the same degree of hypotension as compared to the phenothiazines (Stoelting, 1999). Azaperone is used mainly in swine for its calming effects when mixing weanlings and feeder pigs, to prevent maternal aggression, for transportation, and for obstetrical conditions. Azaperone has also been used as an anesthetic adjunct and sedative in a number wildlife species (Morkel et al., 2010; Radcliffe et al., 2000; Williams et al., 1981; Still et al., 1996). Stresnil, Suicalm, Fluoperidol, Eucalmyl, Sedaperone. 1-(4-fluorophenyl)-4-(4-pyridin-2-ylpiperazin-1-yl)butan-1-one (Figure 14.2). Figure 14.2 Structure of azaperone. 327.4 g/mol. C19-H22-F-N3-O. The water solubility is 131 mg/l. Azaperone produces varying levels of sedation depending on species and dose. Its effects range from behavior modification to chemical restraint (Radcliffe et al., 2000; Nishimura et al., 1993; Clutton et al., 1997; Serrano and Lees, 1976). However, there have been isolated reports of paradoxical excitement following intravenous administration of azaperone in the horse (Dodman and Waterman, 1979). Azaperone causes a decrease in blood pressure, presumably from reduction in systemic vascular resistance from α1-adrenergic blockage (Clarke, 1969). The hypotension may be associated with an increase in heart rate. It is unclear if the increase in heart rate is a response to hypotension or from a central vagolytic effect (Nashan et al., 1984; Serrano and Lees, 1976), or both. The cardiovascular changes lasted longer than the sedative effects of azaperone. Azaperone generally produces mild respiratory depression. Respiratory depression may be enhanced when combined with other CNS depressants. Interestingly, there have been reports of increase in respiratory rates in rats, horses, and pigs (Fish et al., 2011). Azaperone produces muscle relaxation similar to the phenothiazine, acepromazine. Packed cell volume (PCV) decreases following administration. The fall in PCV is presumably secondary to vasodilation, which would result in splenic sequestration of erythrocytes and translocation of fluid into the intravascular space in response to hypotension. Butyrophenones do not interrupt the pain pathway and azaperone did not produce any analgesia in a mechanical nociceptive stimulus model in laboratory animals (Mataqueiro et al., 2004). There is limited information regarding the pharmacokinetics of the butyrophenones in veterinary species. The onset time after intramuscular injection is less than 10 minutes in most species with a peak effect seen approximately 30 minutes after administration (Serrano and Lees, 1976). The duration of action is 2–4 hours in pigs, longer in older pigs. Azaperone in pigs is biotransformed in the liver with 13% excreted in the feces. In rats, 25% of the drug in removed via the kidney and 10% is excreted unchanged (Fish et al., 2011). Metabolite residues are highest in the kidneys and are present in the urine for at least 3 days after administration; however, most of the drug is eliminated from the body with 16 hours (Arneth, 1985). The ability to thermoregulate is decreased in patients administered butyrophenones. The effect on thermoregulation is a combination of α1-receptors blockage (vasodilation) as well as antidopaminergic effects in the thermoregulatory centers in the medulla. Care should be taken when these drugs are administered to patients in cold temperatures as their normal compensatory response of vasoconstricting vessels to conserve heat loss is compromised. Butyrophenones may produce dysphoric effects, especially in patients with a high level of anxiety. Humans describe a feeling of restlessness (akathisia), which may manifest as pacing and agitation in veterinary patients. Extrapyramidal signs are seen in 1% of human patients with the use of droperidol. These droperidol-induced extrapyramidal signs may be treated with diphenhydramine IV (Stoelting, 1999). Muscle tremors, spasticity, and irritability have been seen in the dog after the use of high doses in the range of 11–22 mg/kg IV. Salivation, sweating, muscle tremors, and vocalization has been reported in the horse following intravenous administration of azaperone at a dose of 0.29–0.57 mg/kg (Dodman and Waterman, 1979). Doses of azaperone greater than 1 mg/kg IM may cause penile prolapse in boars, which may predispose to injury. Paraphimosis is presumably secondary to vasodilatory effects. See Box 14.2. Azaperone comes as a clear, pale yellow sterile injectable solution with a concentration of 40 mg/ml in a 100 ml multidose bottle. Azaperone should be discarded 28 days after the multidose vial has been opened. Butyrophenones potentiates the effects of other anesthetic agents (CNS depressants), which will necessitate a reduction in the dose of induction and inhalational agents (Geel, 1991; Bustamante and Valverde, 1999; Nunes et al., 2001; Yamashita et al., 2003). Butyrophenones inhibit the effect of dopamine on renal blood flow (Bradshaw et al., 1980). The use of butyrophenones in patients receiving selegiline may precipitate extrapyramidal movement signs. There are no known antagonists for butyrophenone drugs and treatment for overdoses should focus on supportive care. Patients should be monitored for hypotension and respiratory depression and treated accordingly. Azaperone in a nonscheduled drug. FARAD has no withdrawal times listed for azaperone in pigs. However, a 10-day withholding period prior to slaughter has been recommended. The first benzodiazepine, chlordiazepoxide, was discovered accidently in 1954 by Dr Leo Sternbach. Diazepam (Valium®) is a simplified version of chlordiazepoxide and was marketed in 1963 for anxiety. Although many benzodiazepines (>50) are used in people and animals for behavioral modification, only diazepam, midazolam, lorazepam, and zolazepam will be discussed as sedatives and adjuncts to veterinary anesthesia. The use of benzodiazepines for managing behavior problems in animals is discussed in Chapter 18. Benzodiazepines are sedative-hypnotics due of their propensity to cause anxiolysis, sedation, and an ability to cause sleep. They are classified as minor tranquilizers. A benzodiazepine consists of a benzene ring which is fused to a seven-member diazepine ring (Figure 14.2). All of the clinically useful benzodiazepines and most of the active metabolites contain an aryl substitute at the 5th position of the diazepine ring as well as N-substitutions at the 1st and 4th positions (5-aryl-1,4-benzodiazepine). The hypnotic-sedative action of these drugs is due to the substitution of a halogen or a nitro group at the 7th position of the benzodiazepine structure. Benzodiazepines bind to the benzodiazepine receptor binding site on the gamma subunit of the gamma-aminobutyric acid receptor subtype A (GABAA). Gamma-aminobutyric acid (GABA) is the primary inhibitor neurotransmitter in the CNS. Activation of the benzodiazepine receptor enhances the effects of GABA on the GABAA receptor. GABAA is a large macromolecule, which also contains a number of binding sites for other sedative drug classes such as barbiturates and alcohols. This explains the synergistic effect of these drugs on GABAA-mediated inhibition of the CNS. Activation of the benzodiazepine binding site on GABAA receptors increase the frequency of the opening of the chloride ion channel, leading to hyperpolarization of the postsynaptic neuron (Yeh et al., 1988), producing decreased neuronal transmission. The heterogeneity of the GABAA subunits is in part responsible for the differing clinical actions of the various benzodiazepines (Upton et al., 2001). The highest concentration of GABAA receptors is found in the cerebral cortex, with very few receptor sites found outside the CNS, hence the minimal cardiopulmonary effects of benzodiazepine drugs (Cornick-Seahorn and Seahorn, 1998). Benzodiazepines are used in veterinary medicine as: anticonvulsants (Chapter 17); adjuncts to anesthetic induction agents; skeletal muscle relaxants; and for behavioral modification (anxiolysis and sedation) (Chapter 18). In the healthy patient, behavioral effects are mild, and may be paradoxical as the reduction in inhibitions can result in vocalization, excitement, and dysphoria. Sedation is more reliable in neonates, geriatrics, or ill patients, or as the author would say “very young, very old, or very sick.” In small ruminants benzodiazepines are generally effective at causing sternal recumbency and sedation. A common use for benzodiazepines is to produce sedation; however, when used alone they can cause unpredictable results. This is especially true in healthy individuals. Paradoxical excitement, agitation, vocalization, and dysphoria may be seen after IV or IM administration. Benzodiazepines are commonly combined with other agents such as opioids, α2-adrenergic agonists (see Section Alpha-2-Adrenergic Receptor Agonists), or NMDA receptor antagonists (e.g., ketamine) to provide a more predictable sedation or chemical restraint. Benzodiazepines can by anxiolytic (hence their use in people to treat phobias), but the decrease in inhibitions can result in unpredictable behavior in veterinary patients. The CNS depression produced by benzodiazepines can reduce the requirements for anesthetic induction agents and lower the inhalant needs. Diazepam potentiates the opioid reduction in the MAC of inhalant anesthesia (Hellyer et al., 2001; Seddighi et al., 2011). Benzodiazepines have a favorable profile with regard to cerebral perfusion, which and makes them useful drugs in the face of central CNS disease. They cause a reduction in cerebral blood flow and an even greater reduction in oxygen consumption (Reves et al., 1985; Hoffman et al., 1986). Diazepam caused a reduction in theta, delta, alpha, and beta-frequencies of the electroencephalogram of anesthetized dogs without any changes to the cardiovascular parameters (Court and Greenblatt, 1992). Diazepam, like other benzodiazepines is anticonvulsant (Wauquier et al., 1979; Podell, 1995, 1996). Anticonvulsants are discussed further in Chapter 17. Clinical doses of benzodiazepines cause minimal cardiovascular depression (Jones et al., 1979) and are commonly administered to patients with cardiovascular disease (Harvey and Ettinger, 2007). At clinical doses, both midazolam and diazepam have minimal effect on cardiac output, stroke volume, systemic vascular resistance, or coronary blood flow (Jones et al., 1979). At clinical doses, benzodiazepines may decrease respiratory rate, but rarely affect ventilation and oxygenation (McDonell and Kerr, 2007). However, at higher doses, there can be a dose-dependent respiratory depression and any respiratory depression may be exaggerated when benzodiazepines are combined with other CNS depressants or when administered to debilitated patients (McDonell and Kerr, 2007). Benzodiazepines potentiate the GABA-ergic mediated muscle relaxation of inhibitory neurons at the level of the spinal cord (Elliott, 1976), which produces reliable muscle relaxation. Benzodiazepines are often administered with other anesthetic drugs that do not provide sufficient muscle relaxation alone (e.g., ketamine, etomidate; see Chapter 12). At the clinical doses, midazolam can cause ataxia and in some species is capable of causing recumbency (Platt et al., 2000). The effects of benzodiazepines with nondepolarizing neuromuscular antagonists are unclear. In an in vitro study using cat muscle, midazolam and diazepam were shown to potentiate neuromuscular blockade (Driessen et al., 1987a); however, in humans, midazolam was not shown to change the blockade or rocuronium (Hepağuşlar et al., 2002). Benzodiazepines do not affect the pain pathway and therefore do not produce analgesia. Valium, Apozepam. C16-H13-Cl-N2-O (Figure 14.3). Figure 14.3 Benzodiazepines. 284.7447. 2H-1,4-Benzodiazepin-2-one, 7-chloro-1,3-dihydro-1-methyl-5-phenyl. Diazepam has been used clinically since 1963 and is often the standard against which all other benzodiazepines are measured. Diazepam is a highly lipid-soluble benzodiazepine which has a much shorter duration of action in the dog and cats compared with horses or people (Table 14.1). Table 14.1 Benzodiazepine pharmacokinetics in various species Many of the unwanted side effects of diazepam are caused by the hyperosmotic vehicle, propylene glycol (see below in this section) and therefore large volumes or constant rate infusions should be administered with caution. It may not be compatible with some aqueous IV fluids. Additionally, propylene glycol can result in erratic absorption with any route other than IV (Divoll et al., 1983). Diazepam can administered rectally and is available in suppository form as an anticonvulsant for children (Knudsen, 1979). In dogs, diazepam at 2 mg/kg via rectal suppository did not result in clinically useful plasma levels of diazepam (Probst et al., 2013); however, the active benzodiazepine metabolites were produced in dogs administered the same dose, which indicates good rectal absorption (Papich and Alcorn, 1995). Propylene glycol, the vehicle of diazepam, can cause lysis of red blood cells (RBC), particularly in cats (Christopher et al., 1989). Large volumes or constant rate infusion may cause clinical hemolysis and therefore should be administered with discretion. Diazepam may cause pain when administered intravenously or intramuscularly (Olesen and Hüttel, 1980). The pain is associated with the vehicle, propylene glycol. Propylene glycol vehicles have also been associated with increased risk of thrombophlebitis (Doenicke et al., 1994). Clinical ways to decrease the pain for the patient involve administration in a larger vein or through a running fluid line to provide dilution. Repeated oral administration of diazepam has been associated severe life-threatening hepatic necrosis in cats (Center et al., 1996). No definitive cause has been established, but it is not recommended to use the oral preparation of diazepam in cats (Center et al., 1996). Diazepam administered intravenously is an appetite stimulant, particularly in cats (Mereu et al., 1976) and goats (Miert et al., 1989). The mechanism of action is unknown, but may be associated with decreased inhibitions or serotonergic mechanisms. Anorexia due to GI stasis should not be treated with diazepam since diazepam also slows down gastric emptying (Steyn et al., 1997). See Table 14.1. See Box 14.3. Diazepam is a DEA Schedule IV drug None of the benzodiazepine drugs are intended for food producing animals and there are no established withdrawal times. There are no FDA veterinary approved products. Injectable formulations of diazepam consist of a 5 mg/ml solution with 40% propylene glycol, 10% ethanol, 5% sodium benzoate/benzoic acid buffer, and 1.5% benzyl alcohol as a preservative. The solution is buffered to a pH of 6.2–6.9. Propylene glycol and ethanol allow the diazepam to be dissolved into solution. The cloudy appearance of solutions does not alter the potency of diazepam; however, do not administer if a precipitate forms and does not clear. Versed, Dormicum, Hypnovel, Rocam. C18-H13-Cl-F-N3 (Figure 14.3). 325.7727. 4H-Imidazo(1,5-a)(1,4)benzodia- zepine, 8-chloro-6-(2-fluorophenyl)-1-methyl- Midazolam is a water-soluble benzodiazepine. The chemical structure of midazolam produces both water and lipid solubility based on pH (Kanto, 1985). The pH of marketed midazolam is acidic (pH = 3.5). The low pH stabilizes the diazepine ring and increases water solubility. After administration the pH increases to >4.0 (physiological pH), which causes the diazepine ring to open, increasing the lipid solubility (Kanto, 1985). Therefore at injection the drug is more water soluble but quickly becomes more lipid soluble once in the body. The lack of propylene glycol allows administration IV, IM, and likely SQ, with reliable bioavailability (Schwartz et al., 2013). Midazolam can also be absorbed intranasally (Henry et al., 1998). However, unlike diazepam, midazolam administered rectally does not result in clinically useful plasma concentrations and cannot be recommended (Schwartz et al., 2013). Midazolam is more potent than diazepam, but has similar pharmacodynamics and is often used interchangeably in the clinical setting. The fact that midazolam can be administered via a variety of routes, it does not cause pain on injection (no propylene glycol), and has a lower cost than diazepam has made midazolam more commonly used in veterinary medicine. See Table 14.1. See Box 14.4. The clinical preparations of midazolam are water based (not in propylene glycol) and buffered to a pH of 3.5. Midazolam is compatible with normal saline and lactated Ringer’s solution. Midazolam is a DEA Schedule IV drug. None of the benzodiazepine drugs are intended for food-producing animals and there are no established withdrawal times. There are no FDA veterinary approved products. Midazolam hydrochloride: Each ml contains midazolam hydrochloride equivalent to 1 mg or 5 mg midazolam compounded with 0.8% sodium chloride. The pH is approximately 3 (2.5 to 3.5) and is adjusted with hydrochloric acid and, if necessary, sodium hydroxide. Ativan. C15-H10-Cl2-N2-O2. 321.162. 10-chloro-2-(2-chlorophenyl)-4-hydroxy-3,6-diazabicyclo[5.4.0]undeca-2,8,10,12-tetraen-5-one (Figure 14.3). Lorazepam is a benzodiazepine drug that can be administered orally and parenterally. In humans, lorazepam is the drug of choice for status epilepticus due to its higher binding affinity to the benzodiazepine receptor and elevated brain concentrations (Podell et al., 1998). It achieves therapeutic levels when administered IV but does not when administered rectally (Podell et al., 1998). Intramuscular bioavailability is veterinary species is unknown, but it is well absorbed in people (Wermeling et al., 2001). Injectable lorazepam is supplied in propylene glycol, which can result in pain on administration and hemolysis, similar to diazepam (Cawley, 2001). In veterinary medicine it is uncommonly used as a sedative or anticonvulsant, but its oral bioavailability makes it suitable as an anxiolytic (Sherman, 2008). 0.02–0.1 mg/kg PO BID (Sherman, 2008). Lorazepam is a DEA Schedule IV drug. None of the benzodiazepine drugs are intended for food-producing animals and there are no established withdrawal times. There are no FDA veterinary approved products The vehicle of lorazepam consists of propylene glycol 79%, polyethylene glycol 18–20%, and benzyl alcohol 2% (v/w). Each ml contains 2 mg or 4 mg. zolazepam HCL. C15-H15-F-N4-O (Figure 14.3). 286.3085. Pyrazolo(3,4-e)(1,4)diazepin-7(1H)-one, 4-(2-fluorophenyl)-6,8-dihydro-1,3,8-trimethyl- Zolazepam is also a water-soluble benzodiazepine, but at acid and physiological pH. In the USA, it is only available in combination with tiletamine, an NMDA antagonist (like ketamine), in the anesthetic drug Telazol® (see Chapter 12 for additional information). Little clinical information exists on zolazepam alone. Zolazepam is only available as part of the mixture of Telazol (see Chapter 12 for dosing of that product). Zolazepam is a DEA Schedule III drug. Note, zolazepam is only available as part of the combination drug Telazol, which is a DEA schedule III drug. None of the benzodiazepine drugs are intended for food-producing animals and there are no established withdrawal times. Zolazepam as part of the combination product Telazol® is the only FDA-approved benzodiazepines for us in dogs and cats. Zolazepam is supplied only with tiletamine as a powder that is reconstituted to 5 ml of solution. Each ml contains 50 mg zolazepam (and 50 mg tiletamine). The reconstituted solution has a pH of 2.2–2.8. There have been case reports of cats developing hepatic failure following repeated oral administration of diazepam (see Section Diazepam Hydrochloride). Diazepam is an appetite stimulant, particularly in cats (see Section Diazepam Hydrochloride). In adult horses, and depending on dose, midazolam may not produce sedation (Hubbell et al., 2013) or can cause pacing, paddling, and agitation (Löscher and Frey, 1984). Benzodiazepines are rarely used alone in adult horses for sedation. (See also Chapter 12.) Zolazepam is metabolized at different rates in different species. This is clinically relevant as it is combined with tiletamine in the product Telazol. Therefore recovery (time and smoothness) from Telazol can be very different in different species. There are some species differences in the cerebral uptake of benzodiazepines in various species. In cats and sheep, midazolam is taken up by the cerebrum faster than diazepam; however, the converse is true for pigs (Upton et al., 2001). This is in part due to the lower brain : blood partition coefficient of midazolam when compared with diazepam. In dogs, the free unbound portion of the benzodiazepines correlates well with the concentration of these corresponding compounds to brain tissue levels (Wala et al., 1991). Benzodiazepines undergo liver metabolism, but the pathways (e.g., reduction, glucuronidation) differ based on the drug and species. Additionally, many of the benzodiazepines have a number of have active metabolites such as desmethyldiazepam, oxazepam, and temazepam (Upton et al., 2001), which also varies by drug and species. Desmethyldiazepam is the main metabolite in most species (Wala et al., 1991), and appears to have the most clinical effect due to its long half-life. Approximately 50% of diazepam is metabolized to desmethyldiazepam in the cat (Cotler et al., 1984). Following glucuronide conjugation benzodiazepines are excreted in the urine (Martin et al., 1990); however, cats perform glucuronidation much slower than other species (Driessen et al., 1987b). It is not uncommon to see a transient period of agitation, vocalization, or excitement following parenteral benzodiazepine administration in a number of species. This unwanted effect is seen more commonly in healthy animals, and less commonly in very young, very old, or ill patients. Since, benzodiazepine drugs when used alone can produce unpredictable behavioral effects, they are often administered in conjunction with other CNS depressants to minimize the risk of paradoxical excitation. As an anxiolytic, benzodiazepines can decrease inhibitions. Therefore caution should be used when sedating animals exhibiting fear-induced aggression, as the disinhibition caused by the benzodiazepines may provoke aggressive behavior. See Section Diazepam Hydrochloride. Patients discontinuing a benzodiazepine require their dose to be tapered off to avoid abstinence syndrome from sudden cessation. Long-term use of benzodiazepines does cause physical dependence in the dog (McNicholas et al., 1983) and the use of flumazenil may precipitate abstinence syndrome (tremors, hot foot walking twitches, tonic clonic seizures, and occasional death) (Oliver et al., 2000; Klotz, 1988). The administration of other CNS depressants (e.g., inhalant anesthetics, propofol, opioids, α2-adrenergic agonists) with a benzodiazepine often results in an additive or synergistic CNS depressant effect (Short and Chui, 1991). Therefore dosing of benzodiazepine or other drugs may need to be adjusted. Diazepam is absorbed by the polyvinylchloride (PVC) materials commonly used in IV solution lines, bags, and syringes (Kowaluk et al., 1983; Daniell, 1975), with up to 50% of the drug being absorbed (Ball and Tisocki, 1999). This can be clinically relevant for patients receiving constant rate infusions or if storing diazepam in administration sets. This binding does not occur with hard plastic (e.g., syringes) when used for administration. Benzodiazepines that first undergo oxidation as part of their hepatic metabolism, such as diazepam or midazolam, may have reduced metabolism if coadministered with cimetidine, erythromycin, isoniazid, ketoconazole (KuKanich and Hubin, 2010), propranolol and valproic acid, whereas rifampin will increase the metabolic rate of benzodiazepines (Lam et al., 2003). Clinical signs of acute intoxication can include ataxia/disorientation, CNS depression or agitation, respiratory depression, weakness, tremors, vocalization, tachycardia, tachypnea, or hypothermia. Treatment should include the use of the specific benzodiazepine antagonist, flumazenil (see Section Flumazenil), and supportive care. Flumazenil was introduced in 1987 by Hoffmann-La Roche under the trade name Anexate™. It is the only benzodiazepine antagonist available in the USA. It has also been marketed under the trade name Romazicon, but is currently used as a generic. Flumazenil is a specific and exclusive benzodiazepine competitive antagonist with a high affinity for the benzodiazepine receptor site of the GABAA receptor. Flumazenil has virtually no agonist activity at the benzodiazepine receptor site. C15-H14-F-N3-O3 (Figure 14.4). Figure 14.4 Flumazenil. 303.3 g/mol. 4H-Imidazo(1,5-a)(1,4) benzodiazepine-3-carboxylic acid, 8-fluoro-5,6-dihydro-5-methyl-6-oxo-, ethyl ester. Flumazenil competitively antagonizes the action of benzodiazepines on the benzodiazepine receptor binding site on the GABAA receptor. Activation of the GABAA receptor results in increased chloride conduction and subsequent hyperpolarization of the postsynaptic membrane (decreased neural conduction). Antagonism of this effect results in more active neural conduction. Flumazenil only binds and antagonizes the benzodiazepine binding site of the GABA receptor. Therefore, it does not antagonize the CNS effects of other GABAA receptor sedative-hypnotics at such as propofol or ethanol. Flumazenil antagonizes endogenous benzodiazepine-like substances, which are elevated in human patients with hepatic encephalopathy (Grimm et al., 1988). Flumazenil is indicated for the competitive reversal of benzodiazepine agonists. Flumazenil attenuates the CNS depressant effects of benzodiazepine drugs. Flumazenil reverses the electroencephalographic changes induced by benzodiazepine in the dog and horse (Greene et al., 1992; Keegan et al., 1993; Johnson et al., 2003; Artru, 1989). In people, flumazenil in the absence of a benzodiazepine did not cause CNS excitement (Forster et al., 1993). The benzodiazepine antagonist, sarmazenil, has been shown to be useful in the treatment of hepatic encephalopathy (Grimm et al., 1988); however, flumazenil has been shown minimal effect in reversing the effects of hepatic encephalopathy (Grimm et al., 1988; Meyer et al., 1998). Flumazenil has no direct effect on left ventricular function or coronary hemodynamics observed in human patients (Marty et al., 1991); however, in cats flumazenil administered after diazepam or midazolam antagonized the fall in blood pressure caused by the benzodiazepines agonists (Driessen et al., 1987c). Flumazenil is effective in reversing the muscle relaxation associated with the benzodiazepine agonists. Tidal volume and minute ventilation are generally restored to normal with the use of flumazenil following a benzodiazepine; however, the CO2 response curve of the respiratory center may still depressed (Shalansky et al., 1993). Flumazenil in the absence of a benzodiazepine does not exhibit any respiratory stimulatory effects even at ten times the dose in people (Forster et al., 1993). Activating or blocking the GABAA receptor should not affect the pain pathway. Flumazenil does not affect intraocular pressure in health human volunteers when given alone, but does reverse the decrease in intraocular pressure seen after the administration of benzodiazepines (Artru, 1991). The use of flumazenil in patients who have been treated chronically with benzodiazepine or have received an overdose of tricyclic antidepressants has the potential of precipitating seizures (Spivey, 1992). This phenomenon of benzodiazepine abstinence syndrome has been seen in dogs experimentally (Lheureux et al., 1992). To help reduce the pain or discomfort at the injection site, flumazenil may be diluted or administered into a large vein. Extravasation of flumazenil may result in local tissue inflammation and necrosis (Smith and Volmer, 2005). There is limited information in veterinary species, but in people flumazenil is extensively metabolized by the liver (99%) to the deethylated free acid and glucuronide conjugate (Klotz, 1988; Schlappi et al., 1988), both of which are excreted in the urine. At least three metabolites have been identified, but none of the metabolites of flumazenil appear to be active (Oliver et al., 2000). Although there is pharmacodynamic evidence that flumazenil is effective in veterinary species, there is limited pharmacokinetics information. In humans, the rapid onset of action is due to a rapid uptake and distribution (Klotz and Kanto, 1988). Pharmacokinetic profile in people includes: half-life of 0.7–1.3 hours; apparent distribution volume 0.6–1.6 l/kg; blood clearance of 520–1300 ml/min; and a plasma protein binding of flumazenil of about 40% (Klotz and Kanto, 1988). In dogs, the time for reversal in a model using midazolam-induced respiratory depression with flumazenil was 120 ± 25 seconds IV and up to 310 ± 134 seconds IM (Heniff et al., 1997). Due to the extensive liver metabolism, hepatic diseases can lengthen the elimination phase. Multiple routes of administration are effective for flumazenil in the dog (IV, IM, sublingual, and per rectal), although the IV route produces the fastest effect (Heniff et al., 1997). Endotracheal administration is also effective in people (Palmer et al., 1998). Despite the fact that flumazenil has a high first-pass effect and the oral route is not recommended (Smith and Volmer, 2005), in dogs it is absorbed after oral administration with peak levels at 1 hour and lasting 4 hours (Wala et al., 1988). However, since benzodiazepine antagonists are generally given due to excessive sedation or side effect, the oral route many not be clinically useful. In dogs the dose for benzodiazepine reversal is 0.01–0.04 mg/kg IV, IM, sublingual, endotracheal, or rectal. Repeated doses of flumazenil may be required for the reversal of a benzodiazepine-induced sedation. In horses, the dose for benzodiazepine reversal is 0.01–0.02 mg/kg IV (Kaegi, 1990; Cornick-Seahorn and Seahorn, 1998). Flumazenil in not an FDA scheduled drug. No withdrawal times have been formulated for flumazenil. There are no FDA veterinary approved products. Intravenous preparations of flumazenil are 0.1 mg/ml in a 5-ml vial. Each ml contains 0.1 mg of flumazenil and is compounded with 1.8 mg of methylparaben, 0.2 mg of propylparaben, 0.9% sodium chloride, 0.01% edetate disodium, and 0.01% acetic acid. The pH is adjusted to 4 using hydrochloric acid and/or sodium hydroxide. There is limited information on overdoses in veterinary species. However, administration of flumazenil in the absence of benzodiazepines has not produced CNS excitement or tachypnea. Complications associated with accidental overdose or idiosyncratic reaction might be successfully treated with either a benzodiazepine or GABAA agonist. Alpha-2-adrenergic agonist drugs are popular in veterinary medicine because they produce profound, reliable sedation as well as analgesia. The first α2-adrenergic agonist drug used in veterinary medicine was xylazine. It was developed in Germany in 1962 as a human antihypertensive drug (Greene and Thurmon, 1988) but clinical evaluation identified sedation as a potent side effect and the drug was marketed to veterinarians for that use. The veterinary use of α2-adrenergic agonists was first reported in the late 1960s (Clarke and Hall, 1969), which revolutionized sedation and anesthesia, particularly in large animal patients. Furthermore, α2-adrenergic agonists act synergistically with opioids so are often used as rescue analgesics. Administration of α2-agonists can be parenteral, intravenous, intramuscular, and subcutaneous, as well as by transmucosal, transdermal, and neuraxial routes. This class of drug also popular because the effects can be reversed with the use of α2-adrenergic antagonist drugs (see Section Alpha-2-Adrenergic Antagonists). The pharmacology of adrenergic agonists and antagonists is covered in Chapter 7. Information on basic pharmacology can be found in that chapter. α2-adrenergic agonists are sedatives that also possess significant analgesic properties. Although described as α2-adrenergic receptor agonists, all of the drugs in this category have some agonist effect on α1-adrenergic receptors, and some will bind and activate imidazole receptors. Alpha-2-receptor agonist drugs differ clinically primarily by their differing affinities for α2 or α1 adrenergic receptors, also referred to as the α2 : α1 ratio (Table 14.2). Drugs that are more specific for the α2-receptor will have a high ratio and the drugs that are less specific for the α2-receptor will have a lower ratio. Table 14.2 Ratio of drug selectivity and imidazoline receptor activity Most α2-adrenergic agonists used in veterinary medicine are administered parenterally and are dispensed in varying concentrations (see Section Veterinary Approved Products). The veterinary exception is a newly marketed transmucosal gel marketed for horses. The human product, clonidine, is available in oral, ophthalmic, and transdermal patch formulations. Dexmedetomidine is also approved as a human injection (Precedex). Alpha-2-adrenergic receptors are G-protein receptors and are located both pre- and postsynaptically in the CNS and peripherally. α2-adrenergic receptors are also located extrasynaptically on the vascular endothelium of vessels as well as on platelets. Furthermore, α2-adrenergic receptors have been divided into four subtypes (α2A, α2B, α2C, and α2D). Humans express only subtype A–C, whereas some domestic animals, such as cattle, express the D subtype. The presence and percentage of subtypes varies with species, which likely explains the different pharmacodynamic effects and susceptibility among difference species. In general, the α2A subtype is associated with arousal and awareness in the brainstem and the α2B subtype is associated with mediating vasoconstriction. Cattle have a higher percentage of α2D subtypes, which also appear associated with arousal. Although it has been demonstrated that activation of particular subtypes results in different physiological responses (e.g., analgesia or vasoconstriction), none of the current drugs are subtype specific. The sedative effects of α2-agonists are mediated by presynaptic binding of α2-adrenergic receptors supraspinally, in the locus ceruleus of the pons (Scheinin and Schwinn, 1992). Binding of these presynaptic receptors results in a decrease synaptic release of norepinephrine (NE) (Cormack et al., 2005). Norepinephrine is the primary neurotransmitter of the sympathetic nervous system and decrease in NE results in decreased neurotransmission and decreased arousal (sedation). The analgesic effects of α2-agonists are primarily mediated in the dorsal horn of the spinal cord via decreased release of NE and substance P. However, there is likely also some descending modulation of nociceptive input via the locus ceruleus (Hellyer et al., 2007). The α2-adrenergic agonists also have agonist activity on α1 and α2-adrenergic receptors found in vessel vasculature (primarily α1). Activation of these receptors results in increase in systemic vascular resistance via vasoconstriction of vessels. The α2-adrenergic agonists also bind at nonadrenergic imidazoline receptors (Khan et al., 1999). There are at least three subtypes of imidazoline receptors. Imidazoline-1 are associated with central blood pressure control and may contribute to the drugs cardiovascular effects, although the extent and significance is yet to be fully understood (Khan et al., 1999). Additionally, activation of imidazoline-2 receptors may contribute to the analgesic effects (Head and Mayorov, 2006). The α2-adrenergic agonist drugs are used in veterinary patients to provide sedation, chemical restraint, analgesia, and as an adjunct to anesthetics. Some α2-agonists are also used as emetics, particularly in cats (see Section Gastrointestinal Effects). Alpha-2-adrenergic receptors produce profound, reliable sedation in most veterinary species (see Section Mechanism of Action). However, there are differences in response based on species, drug, and dose. The pharmacodynamic differences between species are likely caused by differences in the α2-adrenergic receptor subtypes in the CNS between species, most notably the presence of α2D-adrenergic receptors in ruminants (Schwartz and Clark, 1998). Ruminants in particular are very sensitive to the sedative effects of certain α2-adrenergic agonists (e.g., xylazine) in the locus coeruleus nucleus (Scheinin and Schwinn, 1992). Therefore, it is likely that the population of α2D-receptor subtypes in the ruminant CNS is different than other animals. The duration and depth of the sedation are dose dependent. Lower doses generally produce mild to moderate sedation, where high doses can produce unconsciousness in some species, such as dogs (Kuusela et al., 2001a, 2003). There does appear to be a ceiling effect for sedation in some species, such as horses, where increased doses generally do not result in recumbency, but rather increases the duration of effect. All currently available α2-agonist drugs have some α1 agonist properties. Activation of only α1-adrenergic receptors in the CNS can cause arousal, agitation, increased locomotor activity, and vigilance (Sinclair, 2003). While excitation is rarely seen, the lack of α1 activation may contribute to why more selective α2-agonist drugs generally produce more reliable sedation (see Table 14.2). Rarely, and surprisingly, sedation with α2-agonists (particularly xylazine, which has the most α1 effect) in horses can result in aggression (Hubbell and Muir, 2004). The CNS depression (and analgesia) associated with α2-agonists produce profound anesthetic-sparing effects (Kuusela et al., 2003; Kauppila et al., 1992; Savola et al., 1991) of both induction agents and MAC sparing of up to 90% (Vickery et al., 1988). It is therefore imperative when using these agents as part of multimodal anesthesia to monitor and adjust other drug doses accordingly. Intracranial pressure was unchanged in dogs anesthetized with medetomidine (Keegan et al., 1995). Dexmedetomidine in anesthetized dogs was associated with a decrease in cerebral blood flow; however, there was no evidence of ischemia even with the reduced cerebral blood flow (Zornow et al., 1990). Although α2-agonists are not associated with increased intracranial pressure and cerebral perfusion was maintained, considerations must include the expected decrease in cardiac output, increase in systemic vascular resistance, as well as the potential for hyperglycemia (see Section Glucose). Furthermore, the high incidence of emesis in susceptible small animals also needs to be considered when used in the preoperative period in animals with elevated intracranial pressure. The use of α2-agonists in patients with CNS disease needs to be assessed on a case to case basis (Cormack et al., 2005). Alpha-2-adrenergic agonists produce a biphasic cardiovascular response. In the “first” phase, activation of presynaptic central α2-adrenergic receptors reduce the sympathetic outflow (decreased norepinephrine) and thereby increases the parasympathetic tone, which will result in negative inotropic, chronotropic, and dromotropic effects on the heart as well as peripheral vasodilation. High vagal tone and decreased dromotropy often causes first and second-degree atrioventricular heart block. Peripherally, postsynaptic α2 and α1-adrenergic receptors are activated in the vascular endothelium causing profound vasoconstriction (which overshadows the vasodilation from the lack of norepinephrine). The increase in arterial blood pressure results in a baroreceptor-mediated reflex bradycardia. Most patients in this first phase are hypertensive and bradycardic. During the “second” phase there is a decrease in systemic vascular resistance (less activation of peripheral vascular adrenergic receptors); however, the heart rate remains low (likely due to decreased norepinephrine). Patients during the second phase may be hypotensive and bradycardic. At therapeutic doses, α2-agonists decrease cardiac output in most species by more than 50% (Pypendop and Verstegen, 1998; Lamont et al., 2001; Bueno et al., 1999). Reduction in cardiac output is not only due to the baroreceptor reflex but concomitant reduction in stroke volume, increase afterload, and low catecholamine levels. There appears to be a ceiling effect with the cardiovascular responses to α2-adrenergic agonists (Kuusela et al., 2000; Pypendop and Verstegen, 1998, 1999). Higher doses produce more sedation, analgesia, and longer duration of action, but do not necessarily worsen the cardiovascular response. The use of anticholinergics prior to or at the same time as an α2-adrenergic agonist is controversial. During the first phase, bradycardia is a protective baroreceptor reflex and generally should not be treated. Treatment with anticholinergics only partially prevent the decrease in cardiac output and increase the risk of dysthymia (Sinclair, 2003; Short, 1991) and hypertension (Singh et al., 1997). Hypertension is more likely when the anticholinergic is administered during the initial vasoconstrictive phase than in the secondary hypotensive phase (Pimenta et al., 2011). Arrhythmias are more likely to occur when the anticholinergic is given after the cardiovascular effects (i.e., hypertension and bradycardia) are seen compared with prior to or concurrently with an α2-agonist (Short, 1991). Arrhythmias associated with α2-agonists and anticholinergics are likely due to increases in myocardial workload (increased heart rate and increased afterload) and oxygen consumption. α1-adrenergic receptors have also been implicated in the formation of arrhythmias mediated via α2-adrenergic agonists, especially with xylazine due to its relatively low α2 : α1-adrenergic receptor affinity (Bozdogan and Dogan, 1999). Alpha-2-adrenergic agonists will redistribute blood flow from nonessential regions such as the skin and viscera to the central organs like the brain, heart, and kidneys (Pypendop and Verstegen, 1998; Lawrence et al., 1996). Furthermore, they do not decrease coronary artery perfusion or myocardial oxygenation (Snapir et al., 2006). This may explain why a class of drugs with so many cardiac effects has been shown to improve survivability in human patients undergoing cardiovascular surgery (Wijeysundera et al., 2003). Alpha-2-adrenergic agonists tend to cause a centrally mediated reduction in respiratory rate and minute ventilation, with no or with mild increases in PaCO2 and mild reductions in PaO2 (Kolliasbaker et al., 1993; Pypendop and Verstegen, 1999; Sinclair, 2003; Lerche and Muir, 2004). The respiratory depression is not as great compared to other anesthetic drugs such as opioids (Sinclair, 2003) or inhalational anesthetics (Bloor et al., 1989). Respiratory depression is exaggerated with the addition of other respiratory depressants or CNS depressants, which can result in hypercapnia, hypoxemia, and cyanosis (Pypendop and Verstegen, 1999). It is speculated that cyanosis of mouth and gums may also be due to slower blood flow through the peripheral capillary beds and an increased oxygen extraction (Sinclair, 2003). It is therefore recommended to monitor patients for hypoventilation and hypoxemia and administer supplemental oxygen as needed, especially in those receiving a combination of respiratory depressants. Serious, species-specific, respiratory side effects caused by α2-adrenergic agonists can occur (Bloor et al., 1989; Celly et al., 1997). The most noted complication occurs in sheep. Within minutes of α2-adrenergic agonist administration in sheep there can be activation of pulmonary intravascular macrophages (PIM) that produce extensive damage to the capillary endothelium and alveolar type I cells, intraalveolar hemorrhage, and interstitial and alveolar edema (Celly et al., 1997). Pulmonary edema causes decreased alveolar gas exchange, an increase in respiratory rate and airway pressures, and a decrease in pulmonary compliance. Hypoxemia is frequently observed and some sheep will die (Celly et al., 1997). While many animal species (e.g., horses) have PIMs, the activation of these by α2-agonists in sheep is more commonly seen clinically. Dexmedetomidine causes similar pulmonary changes in the sheep and goat (Kutter et al., 2006). Treatment with an α2-adrenergic antagonists appears to prevent further activation of PIMs, reverses sedation, and many animals improve clinically. However, the pulmonary effects are not completely eliminated with reversal. Alpha-2-adrenergic agonists produce reliable muscle relaxation, which is mediated via their interaction with the interneurons in the spinal cord (Sinclair, 2003). They are frequently administered for this effect and to balance drugs that do not provide good muscle relaxation (e.g., ketamine). Muscle twitching has been noted in the dog (Sinclair, 2003) and head bobbing and facial twitching has also been observed in the horse (Lloyd Industries, 2014). Alpha-2-agonist drugs can effect muscle tone and contraction of smooth muscle such as the uterus and GI tract (see Sections Gastrointestinal Effects and Reproductive Effects). Alpha-2-adrenergic agonist (xylazine) may cause vomiting in up to 90% of cats and 30% of dogs (Cullen, 1999). It has been theorized that α2-agonists interact with the chemoreceptor zone located in the area postrema to stimulate dopamine and norepinephrine receptors to cause emesis. This appears to be a postsynaptic α2-adrenergic receptor mediated event, which can be antagonized by α2-antagonist drugs (Jovanovic-Micic et al., 1995). Alpha-2-agonists decrease gastrointestinal motility, prolong intestinal transit time, and inhibit colonic motility in a number of species, with the large bowel more sensitive to the effects of α2-adrenergic agonists in ruminants, dogs, and horses (Sasaki et al., 2000; Maugeri et al., 1994). The depression in gastrointestinal motility can be attenuated with the use of an α2-antagonist. α2-agonists also decrease gastric acid secretions. The effects on intestinal motility must be considered when these drugs are used to sedate animals undergoing diagnostic tests of intestinal function. This should also be considered when administered to animals prone to complications from ileus (e.g., horses). Alpha-2-adrenergic agonists cause diuresis by multiple mechanisms. They reduce the production or release of antidiuretic hormone (ADH, arginine vasopressin) from the pituitary (Humphreys et al., 1975; Reid et al., 1979); inhibit the actions of ADH on the collecting tubules; and enhance the excretion of sodium (Gellai and Edwards, 1988; Smyth et al., 1985). Renin levels are decreased by the direct activation of renal α2-adrenergic receptors and indirectly by the initial hypertension produced by the α2-adrenergic agonists (Smyth et al., 1987) further contributing to diuresis. Alpha-2-adrenergic agonists also affect micturition by decreasing micturition pressure, bladder capacity, micturition volume, and residual volume via both spinal and peripherally mediated mechanism (Ishizuka et al., 1996). This, combined with diuresis, will cause animals treated with α2-agonists to produce large amounts of dilute urine that is frequently voided. Predicting the effects of α2-adrenergic agonists on catecholamines and cortisol levels is difficult. In general, α2-adrenergic agonists will decrease catecholamine due to their presynaptic effects on the release of norepinephrine. However, the effects on basal or induced cortisol varies based on drug, dose, and species. In the horse, detomidine will suppress catecholamine activity without suppressing cortisol activity (Raekallio, 1991) whereas in cattle, medetomidine will suppress catecholamine activity without suppressing cortisol activity (Ranheim, 2000). Interestingly, in the dog, high doses (80 μg/kg) of dexmedetomidine suppressed cortisol levels as well as prevented stimulation with ACTH (Maze et al., 1991). However, at clinical doses, dexmedetomidine did not change cortisol level in dogs (Restitutti et al., 2012). The effect of α2-adrenergic agonists on glucose homeostasis is complex and dependent upon the drug, the dose, and the species. Hyperglycemia reported after the use of α2-adrenergic agonists is likely due to a decrease in insulin release from the β-cells in the pancreas and/or increase glucagon release from the α-cells (increased gluconeogenesis) (Angel et al., 1990; Niddam et al., 1990). The α2-adrenergic agonist, xylazine, causes a mild transient hyperglycemia in cows and horses (Thurmon et al., 1984; Hsu and Hummel, 1981). Medetomidine causes a decrease in insulin without the resultant increase in glucose in dogs (Ambrisko and Hikasa, 2003). Clinical hyperglycemia secondary to α2-adrenergic agonist administration is generally not high enough to pass the renal threshold for glucose (∼180 mg/dl) and therefore should not produce glucosuria. Alpha-2-agonist drugs can effect myometrial tone as well as myometrial contraction; however, these effects are related to species, dose, time of reproductive cycle, and species. Furthermore, the clinical effect of α2-adrenergic agonists on uterine contractility and blood flow is influenced by the level of estrogen and progesterone. Estrogen leads to an up-regulation in α-adrenergic receptors while progesterone will cause a down-regulation due to changes in the transmembrane signal pathways that cause the differences in myometrial contractility (Re et al., 2002). Myometrial contractions in the nongravid uterus were observed at any equipotent dose of α2-adrenergic agonists (Schatzmann et al., 1994; Jedruch et al., 1989) and there is a case report of cows having premature labor after the administration of xylazine (Vanmetre, 1992). Lower doses of detomidine (<60 μg/kg IM) in the cow and horse, and medetomidine (<20 μg/kg IV) in the dog, decreased myometrial contractions (Jedruch and Gajewski, 1986; Jedruch et al., 1988, 1989). Higher doses of medetomidine in the dog did cause an increase in myometrial contraction (Jedruch et al., 1989). This biphasic response in the gravid uterus to α2-adrenergic agonists may possibly be mediated by their effect on the α1-adrenergic receptor (Ford, 1995; Gaspar et al., 2001; Jedruch et al., 1989). The effects of medetomidine (40 μg/kg IM) and xylazine (200 μg/kg IM) on intrauterine pressure and uterine blood flow have been studied in the goat (Sakamoto et al., 1996, 1997). Both agents crossed the placenta, reduced uterine blood flow for 120 minutes, and increased intrauterine pressure. These observations would suggest α2-adrenergic agents should be used with caution in near-term pregnant animals, especially if there are historical or physical evidence to suggest fetal distress is already present. Patients may be unable to normally control body temperature in response to the administration of α2-adrenergic agonists. This is due to the combination of CNS depression, reduction in muscle activity (reduction in shivering), and loss of vasomotor control. Clonidine and dexmedetomidine have been shown to decrease the vasoconstrictive and shivering thresholds (Talke et al., 1997). Animals may be cool to touch and unable to thermoregulate their body temperature. Care must be taken during the recovery phase to prevent both cooling and overheating of these animals. The use of α2-adrenergic agonists during the postoperative period following inhalant anesthesia of horses has been shown to improve the quality of the recovery and reduce the number of attempts to stand, at the expense of slightly prolonging the recovery period (Bienert et al., 2003; Santos et al., 2003). The dose of α2-adrenergic agonists used during this postanesthesia period may be as little as 10–20% of the “usual” premedication dose (Santos et al., 2003). Romifidine when used as a premedicant only exhibited a better quality of recovery as compared to xylazine (Jaugstetter et al., 2002), although recovery quality must be assessed in relation to the other anesthetic drugs administered, procedure performed, and recovery environment. The use of intraoperative constant rate infusions of medetomidine and romifidine have been shown to improve the quality of the recovery in horses with fewer attempts to stand (Kuhn et al., 2004). At very high doses, α2-adrenergic agonists enhance catecholamine-potentiated platelet aggregation (Sjoholm et al., 1992). The clinical consequences of this reaction has not been documented. Alpha-2-agonists produce profound analgesia and can be administered parenterally and neuraxially (e.g., epidurally). The first report of α2-adrenergic agonist-induced analgesia was in 1974 evaluating clonidine as an analgesic in rats (Paalzow, 1974). Analgesia is produced by activation of α2-receptors in the CNS in the locus coeruleus (Guo et al., 1996; Schwartz and Clark, 1998) and in the substantia gelatinosa of the dorsal horn of the spinal cord (Savola and Savola, 1996). The effects in the brain are primarily by decreased neural conduction, whereas the effects in the spinal cord are primarily through decreasing release of norepinephrine and substance P (see Section Mechanism of Action). Some of the analgesia may be mediated via I2-imidazoline receptors (Diaz et al., 1997; Regunathan, 2006) as imidazoline receptor agonist and antagonists can modulate the analgesia produced by morphine (Gentili et al., 2006). The analgesia produced by α2-agonists is synergistic with opioids, lidocaine, and N-methyl-D-aspartate (NMDA) receptor antagonists (e.g., ketamine) (Glynn and O’Sullivan, 1996; Lee and Yaksh, 1995; Regunathan, 2006). Therefore these drugs are often used in conjunction with other analgesics. In people with tolerance to opioids or chronic pain syndromes, α2-adrenergic agonists are routinely used for rescue analgesia. A major limiting factor to use of this class for analgesia is the concurrent sedation and ataxia. Interestingly, higher plasma levels are needed to produce analgesia than sedation, with horses needing 10 times the plasma level to produce visceral nociception compared with plasma levels needed to produce sedation (Elfenbein et al., 2009). Epidural administration can produce potent analgesia with minimal sedative or cardiovascular effects compared with intravenous administration (Aminkov and Pascalev, 1998; Greene et al., 1995). The primary receptor subtypes found in the spinal cord are α2A and α2C, but α2A-receptors appears to most active in the nociceptive pathway (Stone et al., 1998). Due to the high lipophilicity of these drugs, there is some systemic absorption and systemic effect (e.g., sedation) after neuraxial administration. The use of α2-adrenergic antagonists following neuraxially administered α2-agonists can reduce the side effects whilst maintaining a good level of analgesia (Skarda, 1991). Clinicians should also note that when α2-agonist reversal agents are administered (α2-antagonists; see Section Alpha-2-Adrenergic Antagonists), to reverse sedation, the analgesic effects will be reversed also. Pharmacokinetics of α2-agonist can vary based on species, drugs, and dose. In general, the onset of action of most α2-adrenergic agonists following intravenous administration is rapid (within minutes) and peak effect usually occurs within 10–15 minutes (Garcia-Villar et al., 1981; Pypendop and Verstegen, 1998). Intramuscular administration of α2-adrenergic agonists will approximately double the time to peak effect; however, the bioavailability is variable (40–95%) depending upon the species (Garcia-Villar et al., 1981; Kästner et al., 2003). Bioavailability of intramuscular xylazine in the horse and sheep is approximately 50% as compared to 75% in the dog (Garcia-Villar et al., 1981). Bioavailability of intramuscular detomidine is variable depending on species: horse 66%, and cow 85%(Salonen, 1989). Bioavailability of intramuscular medetomidine is close to 100% in dogs and cats (Salonen, 1989). The pharmacokinetic parameters of the various α2-adrenergic agonists in the common domestic species are summarized in Table 14.3. Table 14.3 Alpha-2-adrenergic agonists pharmacokinetics Interestingly, there is a lack of correlation between plasma levels and pharmacodynamic effects of medetomidine in cows and sheep (Ranheim et al., 1999, 2000). Clinical effects can be seen for up to 7 hours despite the plasma levels being detectable for only 2 hours. This discrepancy implies either a long tissue binding period or an active metabolite (Ranheim et al., 1999, 2000). Equipotent intravenous doses for sedation in the horse are considered to be the following: xylazine (1 mg/kg), medetomidine (5–10 μg/kg), romifidine (40–80 μg/kg), and detomidine (20–40 μg/kg) (England et al., 1992; Yamashita et al., 2002). The order in which they are listed also demonstrates the duration of sedation from the shortest to longest in duration (Freeman and England, 2000; Hamm et al., 1995; Yamashita et al., 2002). Detomidine tends to cause the longest duration of ataxia (Hamm et al., 1995; England et al., 1992). There appears to be less ataxia and lowering of the head with romifidine as compared to the other α2-adrenergic agonists; however, the level of analgesia achieve may be comparable to (England et al., 1992; Spadavecchia et al., 2005) or lower than (Moens et al., 2003; Hamm et al., 1995) other α2-adrenergic agonists, depending upon the stimulus used. The apparent order of ataxia in horses produced by these agents from least to most appears to be: romifidine < xylazine < medetomidine and detomidine. Rompun AnaSed, Cervizine. C12-H16-N2-S (Figure 14.5). Figure 14.5 Alpha-2-agonists. 220.338 g/mol. N-(2,6-dimethylphenyl)-5,6-dihydro-4H-1,3-thiazin-2-amine (XX). Xylazine was the first α2-adrenergic agonists used in veterinary medicine and its clinical use was first described in the 1969 in a number of species (Clarke and Hall, 1969; Keller, 1969; Müller et al., 1969). Xylazine is often thought of as the prototypical α2-adrenergic agonist; however, it does have one of the lowest α2 : α1 affinities (Table 14.2), and does not bind to imidazoline receptors. It is still the most commonly used α2-agonist in horses and is also commonly used in lab animal and wildlife medicine. Interestingly, although rare, xylazine has been associated with aggressive behavior following administration in horses (Hubbell and Muir, 2004), which may be due to the excitatory effects of α1-agonists in the CNS (see Section Central Nervous System Effects). Cattle are particularly sensitive to the effects of xylazine and require a much reduced dose compared with other species and approximately one-tenth the dose compared with horses. The dose rate of other α2-adrenergic agonists in cattle is similar to other species. Conversely, swine require two to three times the dose of xylazine compared to the dog, cat, or horse. These species differences are likely due to differences in G-protein signaling pathways (Törneke et al., 2003), as well as anatomical differences in α2 and α1-receptor distributions (Hellyer et al., 2003). Xylazine is the α2-agonist most likely to cause vomiting in the dog, whereas almost all α2-agonists cause vomiting in cats (Jovanovic-Micic et al., 1995; Sinclair, 2003). See Box 14.5.
Sedatives and Tranquilizers
Introduction
Phenothiazine Derivatives
Introduction
Chemistry
Classification:
Mechanism of Action
Indications
Physiological Effects
Central nervous system effects:
Cardiovascular effects:
Respiratory effects:
Musculoskeletal effects:
Pharmacokinetics and Metabolism
Miscellaneous
Equine recovery from anesthesia:
Thermoregulation:
Platelet aggregation:
Hematocrit:
Antiemetic:
Phototoxicity:
Antihistamine:
Analgesia:
Specific Drugs
Acepromazine Maleate
Synonym:
Systematic chemical name:
Molecular formula:
Molecular weight:
How supplied:
Dosage for acepromazine:
Promazine Hydrochloride
Synonym:
Name:
Molecular formula:
Molecular weight:
How supplied:
Dosage for promazine granules:
Chlorpromazine Hydrochloride
Chemical name:
Molecular formula:
Species Differences
Horse:
Dog:
Pig:
Drug Interactions
Overdose/Acute Toxicity
Contraindications
Regulatory Information
Controlled drug status:
Withdrawal times:
Veterinary Approved Products (Representative)
Acepromazine
Promazine
Chlorpromazine
Butyrophenone Derivatives
Introduction
Chemistry
Classification:
Mechanism of Action
Indications
Azaperone
Synonym:
Systematic chemical name:
Molecular weight:
Molecular formula:
Pharmacodynamic Effects
Central nervous system effects:
Cardiovascular effects:
Respiratory effects:
Musculoskeletal effects:
Miscellaneous:
Analgesia:
Pharmacokinetics Properties
Adverse Effects/ Contraindications
Thermoregulation:
Dysphoria and akathisia:
Paraphimosis:
Representative Veterinary Dosages
How supplied:
Drug Interactions
Overdose/Acute Toxicity
Regulatory Information
Controlled drug status:
Withdrawal times:
Veterinary Approved Products (Representative)
Benzodiazepine Derivatives
General Pharmacological Considerations
History/ Introduction
Classification
Chemistry:
Mechanism of Action
Indications
Pharmacodynamic effects
Central nervous system effects:
Cardiovascular effects:
Respiratory effects:
Musculoskeletal effects:
Analgesia:
Specific Drugs
Diazepam Hydrochloride
Synonyms:
Molecular formula:
Molecular weight:
Chemical name:
Drug
Species
Dose (mg)
Volume of distribution (l/kg)
Protein binding (%)
Clearance (ml/kg/min)
Elimination half-life (minutes)
Reference
Diazepam
Dog
0.5 mg/kg IV
14–16
(Papich and Alcorn, 1995)
Dog
2.0 mg/kg IV
192
(Löscher and Frey, 1981)
Cat
5.0 mg/kg IV
4.72 ± 2.45
330
(Cotler et al., 1984)
Horses
0.2 mg/kg IV
210–1320
(Muir et al., 1982)
Midazolam
Dog
0.5 mg/kg IV
3.0 ± 0.9
96
59–95
(Court and Greenblatt, 1992)
Dog
0.2 mg/kg IV
1.1 ± 0.68
10.1 ± 1.9
63.3 ± 28.5
(Schwartz et al., 2013)
Horse
0.1 mg/kg IV
2.8 (2.2–7.0)
10.4 (8.4–17.6)
408 (192–924)
(Hubbell et al., 2013)
Lorazepam
Dog
0.3 mg/kg IV
100 ± 15 l
(Podell et al., 1998)
Hemolysis:
Pain on administration:
Hepatic necrosis in cats:
Appetite stimulation:
Pharmacokinetic Data
Representative Veterinary Dosage
Regulatory Information
Controlled drug status:
Withdrawal times:
Veterinary Approved Products
How supplied:
Midazolam Maleate
Synonyms:
Molecular formula:
Molecular weight:
Chemical name:
Pharmacokinetic Data
Representative Veterinary Dosages
How Supplied
Regulatory Information
Controlled drug status:
Withdrawal times:
Veterinary Approved Products
How supplied:
Lorazepam
Synonym:
Molecular formula:
Molecular weight:
Chemical name:
Representative Veterinary Dosages
Dog for anxiolytic effects:
Regulatory Information
Controlled drug status:
Withdrawal times:
Veterinary Approved Products
How supplied:
Zolazepam
Synonym:
Molecular formula:
Molecular weight:
Chemical name:
Representative Veterinary Dosages
Regulatory information
Controlled drug status:
Withdrawal times:
Veterinary Approved Products
How supplied:
Species Differences
Cat:
Horses:
Zolazepam and veterinary species:
Cerebral uptake of benzodiazepines:
Metabolism
Adverse Effects/ Contraindications
Paradoxical excitation
Behavioral disinhibition
Hepatic Necrosis in Cats with Oral Diazepam
Addiction/Physical Dependence
Drug Interactions
Overdose/Acute Toxicity
Benzodiazepine Antagonists
General Pharmacological Considerations
History/ Introduction
Classification
Specific Drugs
Flumazenil
Molecular formula:
Molecular weight:
Systematic chemical name:
Mechanism of Action
Indications
Pharmacodynamic Effects
Central nervous system effects:
Cardiovascular effects:
Musculoskeletal effects:
Respiratory effects:
Analgesia:
Miscellaneous:
Adverse effects/ contraindications
Metabolism
Pharmacokinetics Properties
Doses
Regulatory Information
Controlled drug status:
Withdrawal times:
Veterinary Approved Products
Veterinary Approved Products
Overdose/Acute Toxicity
Alpha-2-Adrenergic Receptor Agonists
Introduction
Classification
Chemistry
α2 : α1
I2-Imidazoline
Compound
selectivity
activity
Agonists
Xylazine
No
Detomidine
Yes
Romifidine
Yes
Medetomidine
Yes
Dexmedetomidine
Yes
Antagonists
Tolazoline
Yes
Yohimbine
No
Atipamezole
No
How Supplied
Mechanism of Action
Indications
Physiological Effects
Central Nervous System Effects
Cardiovascular Effects
Respiratory Effects
Musculoskeletal Effects
Gastrointestinal Effects
Emesis:
Motility and acid secretion:
Renal effects
Neuroendocrine Stress Response
Glucose
Reproductive Effects
Miscellaneous
Thermoregulation:
Equine recovery:
Platelet activation:
Analgesia
Pharmacokinetics Properties
Drug
Species
Dose (mg/kg)
Mean Residence Time (minutes)
Volume of distribution (L/kg)
ClearanceTot (mL/kg/min)
Elimination half-life (mins)
Reference
Xyalzine
Dog
1.4 (IV)
2.52
81
30.1
(Garcia-Villar et al., 1981)
Cat
Horse
0.6 (IV)
2.46
21
49.5
(Garcia-Villar et al., 1981)
Cattle
0.2 (IV)
1.94
42
36.5
(Garcia-Villar et al., 1981)
Sheep
1.0 (IV)
2.74
83
23.1
(Garcia-Villar et al., 1981)
Detomidine
Horse
0.08 (IV) [IM]
0.74 ± 0.25 [1.56]
7.1 ±1.6 [10.1]
71.4 ± 16.2 [106.8]
(Salonen et al., 1989)
Cattle
0.08 (IV) [IM]
0.73 ± 0.17 [1.89]
9.5 ± 1.9 [12.3]
79.2 ± 27 [153.6]
(Salonen et al., 1989)
Medetomidine
Dog
0.04 (IV)
1.28 ± 0.19
21.0 ± 7.3
57 ± 15
(Kuusela et al., 2000)
Dog
0.08 (IV)
2.8
31.5
58.2
(Salonen, 1989)
Dog
0.08 (IM)
3.0
27.5
76.8
(Salonen, 1989)
Cat
0.08 (IM)
3.5
29.5
81
(Salonen, 1989)
Horse
0.007 (IV)
32.9 ± 7.21
2.2 ± 0.52
66.6 ± 9.9
51.3 ± 13.09
(Bettschart-Wolfensberger et al., 1999)
Calf
0.04 (IV)
30.1 ± 4.0
1.75 ± 0.30
33.1 ± 5.5
44.4 ± 14.2
(Ranheim et al., 1998)
Lactating dairy
cows
0.04 (IV)
72.7 ± 30.7
1.21 ± 0.32
24.2 ± 6.5
52.7 ± 25.3
(Ranheim et al., 1999)
Sheep
0.04 (IV)
37.2 ± 10
1.7 ± 0.26
44.2 ± 11.3
34.8 ± 7.3
(Ranheim et al., 2000)
Sheep
0.03 (IM)
70 ± 17.4
3.9 ± 2.4
81.0 ± 21.5
32.7 ± 14.9
(Kastner et al., 2003)
Dexmedetomidine
Dog
0.02 (IV)
0.86 ± 0.22
20.1 ± 8.0
47 ± 14
(Kuusela et al., 2000)
Young Ponies (aged)
0.0035 (IV)
19.8 (28.9)
(Bettschart-Wolfensberger et al., 2005)
Levomedetomidine
Dog
0.02 (IV)
2.68 ± 0.57
67.8 ± 11.5
38 ± 18
(Kuusela et al., 2000)
Clonidine
Sheep
0.006 (IV)
∼ 5L
95
(Castro and Eisenach, 1989)
Comparative Equine Doses and Effects
Specific Veterinary Drugs
Xylazine hydrochloride
Synonyms:
Molecular formula:
Molecular weight:
Systematic chemical name:
Dosage
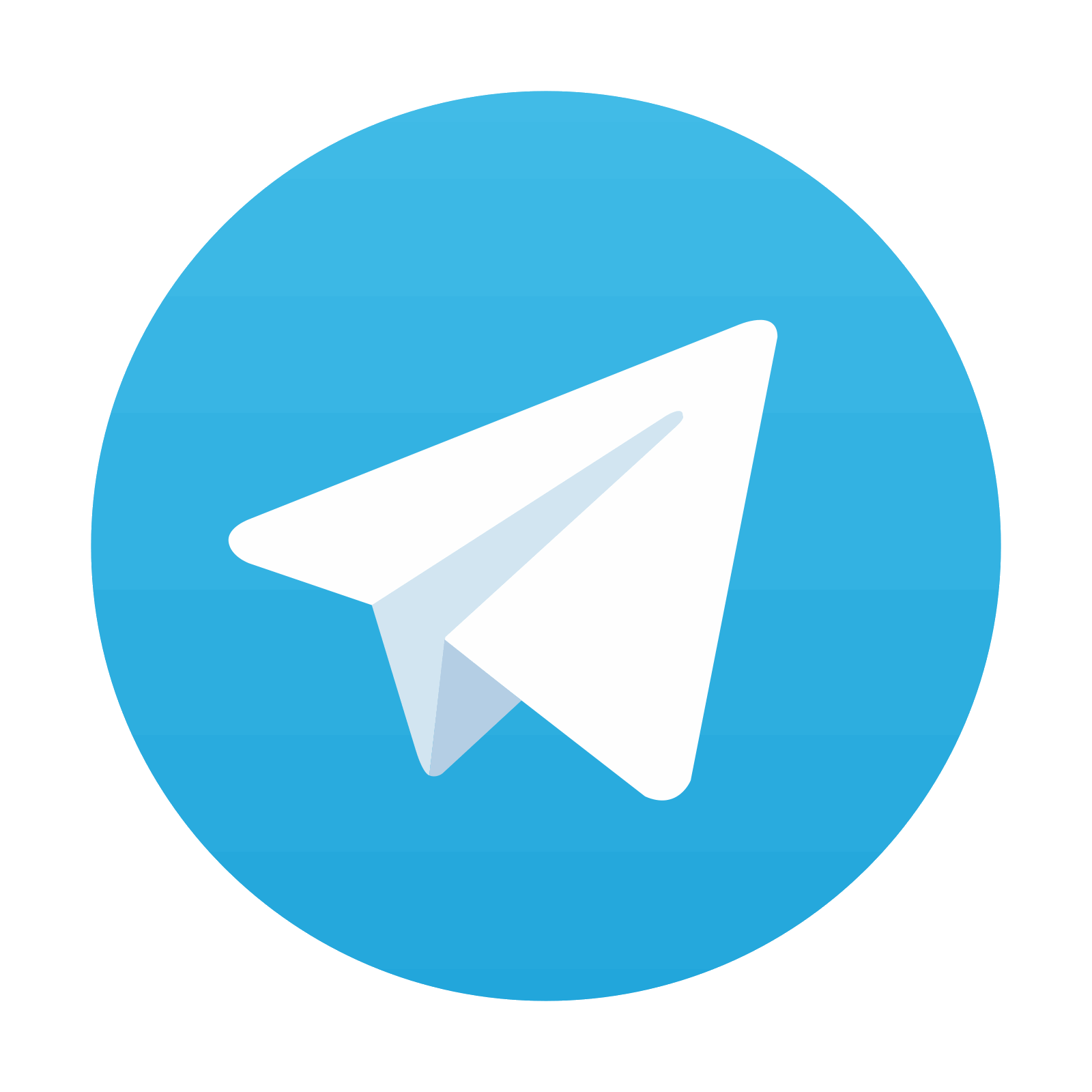
Stay updated, free articles. Join our Telegram channel
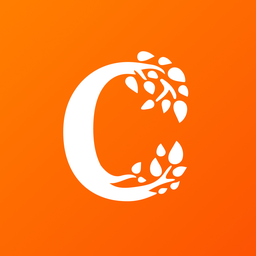
Full access? Get Clinical Tree
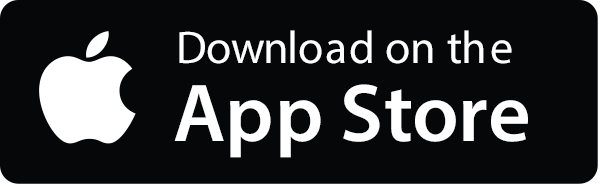
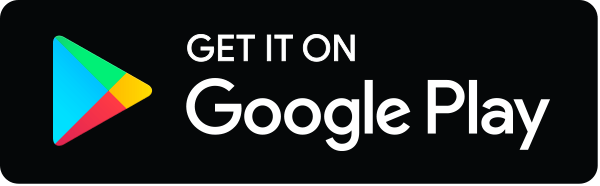