Paul A. Barrow, Michael A. Jones, Kate C. Mellor and Nick R. Thomson Salmonella are Gram‐negative bacilli belonging to the family Enterobacteriaceae. They are motile, non‐spore‐formers and facultatively anaerobic. There are two species: Salmonella enterica and Salmonella bongori. S. enterica is the type species, and is divided into six subspecies. The most important, from the point of view of human and animal disease, is S. enterica subsp. enterica which includes over 2600 serotypes in the White–Kauffman–LeMinor scheme. A small number of serotypes/serovars typically produce typhoid‐like infections in a relatively narrow range of host species, including S. enterica subsp. enterica Typhi (S. Typhi) and S. Paratyphi in humans, S. Choleraesuis in pigs, S. Dublin in cattle, S. Gallinarum and S. Pullorum in poultry, S. Abortusovis in sheep and S. Abortusequi in horses. The remaining serovars are associated more with the production of gastroenteritis in humans and young livestock. The current classification of Salmonella is extremely complex. Based on DNA–DNA hybridization results, the genus Salmonella is divided into two major species: S. enterica and S. bongori. S. enterica is then further divided into six distinct subspecies (I, II, IIIa, IIIb, IV, and VI), based on biochemical differences or genomic population structure, which is indicative of novel subspecies (Figure 8.1). The speciation history of the genus may have involved speciation by hybridization or multiway speciation events. Isolates from many serovars, including Typhimurium, Typhi, and Enteritidis, show a conserved genomic signature and so these serovars are considered monophyletic. However, other Salmonella serovars show significant genome variation between isolates, and, despite being co‐classified by serology, the lineage is considered to be polyphyletic in nature (i.e. not all of the isolates of the same serovar show the same genomic signature). Serovars that appeared to be polyphyletic include Dublin, Enteritidis, Infantis, Muenster, Paratyphi B and Saint Paul (Langridge et al. 2015). Analysis has also confirmed previous notions that there are close genetic relationships between some serovars, such as between S. Enteritidis and S. Dublin or S. Paratyphi C and S. Choleraesuis Figure 8.1. Features of the S. enterica subsp. enterica serovar genomes, which were the first to be sequenced, are shown in Table 8.1; the serovars have similarly sized genomes and gene numbers. Alignment of the whole‐genome sequences of these isolates shows that the genomes are remarkably conserved, displaying a high degree of overall synteny; all the genomes being essentially colinear except for insertions and several large‐scale rearrangements symmetrically around the origin or terminus of replication. This is the most common form of chromosomal rearrangement separating related bacterial genomes. The reciprocal inversion event seen in S. Typhi is well documented and is the result of recombination between rRNA operons, which are the most likely sites for recombination to occur (Tillier and Collins 2000). Figure 8.1 Grape‐tree representation of a maximum likelihood phylogeny of core single‐nucleotide polymorphisms from 926 representative genomes of Salmonella enterica plus Salmonella bongori. Source: Alikhan et al. (2018) PLOS/CC BY 4.0. Interestingly, if the genomes of these Salmonella serovars are aligned with E. coli strain K12, for example, a high degree of collinearity is still maintained even after a separation estimated at 100–140 million years (Doolittle et al. 1996). This is consistent with the notion that the enteric genome architecture was fixed early in enteric evolution, prior to the adaptation to different hosts seen in Salmonella, and has been actively maintained. In whole‐genome comparisons of S. Typhi and S. Typhimurium, the core genome comprises around 87% of its coding capacity (McClelland et al. 2001; Parkhill et al. 2001). The core genome is thought to encode essential metabolic and colonization functions that facilitate the general enteric lifestyle. The core regions are interspersed with regions that are either unique to that isolate or of restricted phylogenetic distribution, termed the accessory genome, with these accessory regions enriched for functions that could explain host range and pathogenic potential of the different Salmonellae. Table 8.1 General properties of Salmonella enterica subsp. enterica serovar genomes. Much of the accessory genome is composed of clusters of three genes or more. The largest individual accessory gene clusters are represented by integrated prophage and Salmonella pathogenicity islands (SPIs), which in S. Typhi range from around 10 to 130 kb. However, less visible are the large number of small gene insertions/deletions. When comparing S. Typhi with S. Typhimurium, there are 145 small insertions/deletions of 10 or fewer genes compared with 12 events of 20 genes or more. Interestingly, there are very few (42) single gene insertions/deletions that are unique to S. Typhi compared with S. Typhimurium. Many of the smaller insertions/deletions of fewer than 10 genes encode functions such as toxins or adhesins, but more commonly these regions encode chaperone–usher fimbrial operons, sugar transport/metabolism systems, and restriction‐modification systems. The genomes of S. Enteritidis, S. Gallinarum and S. Pullorum resemble each other more than they do S. Typhimurium in terms of shared genes and pairwise sequence similarity. It was postulated that this was because the common S. Enteritidis strains isolated from animals and humans were common ancestors for the avian‐adapted serovars. However, genomic analysis has revealed important nuances in our understanding of the evolutionary biology of these and other Salmonellae. For example, S. Gallinarum and S. Pullorum did not directly evolve from contemporary S. Enteritidis, as had been previously thought (Thomson et al. 2008). All three serovars are adapted lineages that have emerged from a more ancient S. Enteritidis‐like predecessor, possibly under anthropogenic pressures such as intensive farming (see further speculation on this elsewhere in this chapter), which, although rarely isolated in humans or farmed animals, could still be found in wild animals (Langridge et al. 2015). Furthermore, the emergence of invasive non‐typhoid Salmonella (iNTS) serovars Typhimurium and Enteritidis has been observed. This recent phenomenon was linked to spread within human populations immunocompromised by malnutrition, severe malaria, or advanced HIV in sub‐Saharan Africa. These iNTS isolates tend to cause acute febrile disease, are often multidrug resistant, and are rarely associated with systemic disease in immunocompetent individuals (Kingsley et al. 2009). Going back in evolutionary time, the majority of the horizontally acquired genes in the Salmonella genomes are likely to have been acquired early in their evolution (see also Chapter 2). S. enterica genes are shared among most of the lineage; 60–70% of the putative horizontally acquired genes, including many key SPI, were inserted following the divergence of Salmonella from the E. coli lineage but prior to the divergence of the S. enterica subspecies. For example, the cob operon of S. enterica encoding vitamin B12 biosynthesis was acquired horizontally in the Salmonella lineage after divergence from E. coli (Lawrence and Roth 1996). The cob genes are thought to have been lost from the enterics early in their evolution and to have been reacquired by Salmonella around 71 million years ago following the split of the S. arizonae and S. enterica lineages (Lawrence and Roth 1996). The difference in the extent of phage‐related functions in S. Typhi and S. Paratyphi A from S. Typhimurium, S. Enteritidis, and S. Gallinarum suggests that recent evolutionary time has been dominated by the acquisition of bacterial viruses (prophage) but that earlier evolutionary events in Salmonella were dominated by the acquisition of metabolic and virulence functions (Vernikos et al. 2007). Horizontally acquired DNA sequences that contain functionally related genes with limited phylogenetic distribution (i.e. present in some bacterial genomes while being absent from closely related ones) are often referred to as pathogenicity islands or genomic islands. These mobile elements are often inserted alongside tRNA genes and have direct repeats and mobility genes (e.g. integrase, transposase), which has led to a definition of the genomic island structure that includes these features (see also Table 2.1; Hacker et al. 1997; Hacker and Kaper 2000; Schmidt and Hensel 2004). Traditionally, genomic islands in Salmonella that carry genes associated with virulence are termed SPIs. SPIs are predicted or known to encode a range of functions including exopolysaccharide synthesis, type III secretion systems () and their associated effector proteins, type four secretion systems, and metal uptake systems. In addition, proteins of unknown function are encoded. It is apparent that most SPIs were acquired early in the evolution of S. enterica, being present in all sequenced isolates. However, there are exceptions to this: SPI‐7, SPI‐8 and SPI‐15 appear to be more recent acquisitions. SPI‐7, for example, is restricted to S. Typhi and some isolates of S. Paratyphi C and S. Dublin (Pickard et al. 2003). Unusually, inserted within SPI‐7 is a prophage ΦSopEST (33.5 kb in size) encoding the SPI‐1 effector protein, SopE, which is important for Salmonella invasion (Wood et al. 1996; Mirold et al. 1999; Friebel et al. 2001). This prophage element probably represents an independent horizontal gene transfer event in S. Typhi, given that it is absent from SPI‐7 in S. Dublin and S. Paratyphi C (Pickard et al. 2003). Many SPIs lack identifiable mobility genes and repeat elements flanking their boundaries. These are probably mobile elements that have stably integrated in the Salmonella chromosome and may be ancient insertions which have, over time, lost their ability to mobilize. Together with the acquisition of genes, prophage, and genomic islands, gene loss has had, and continues to have, an important role in the fluidity of the Salmonella genome and resulting host adaptations. Pseudogenes are defined as being untranslatable due to the presence of stop codons, frameshifts, internal deletions, or disruption following the insertion of an insertion sequence. There are always dangers to in silico prediction of pseudogenes and from assuming that a gene function has been lost when a significant part of the coding sequence has been deleted. The enrichment of functionally significant mutations in genes has been associated with a shift in the pathogenic niche. Degradation of metabolic pathways has been identified in S. Enteritidis and Typhimurium lineages associated with invasive disease in immunocompromised humans and highly virulent bird‐associated S. Typhimurium strains (Langridge et al. 2015). The dramatic expansion of insertion‐sequence element numbers and subsequent recombination between these elements is thought to be the result of a recent evolutionary bottleneck: conditions thought to increase the fixation of mutations by genetic drift and associated with a switch in lifestyle (Andersson and Hughes 1996). These observations were made for S. Typhi and have been suggested to be a marker of host restriction and adaptation to humans. Similar observations were made for S. Gallinarum, which is closely related to S. Enteritidis but is restricted to infecting galliform birds following extensive degradation, including metabolic genes required for survival in the intestine (Thomson et al. 2008; Langridge et al. 2015). S. Gallinarum causes a typhoid‐like systemic infection in Galliformes, unlike S. Enteriditis, which is usually associated with self‐limiting enterocolitis. Analysis of the S. Typhi genome revealed over 200 pseudogenes, while S. Typhimurium was predicted to contain only around 39 (McClelland et al. 2001; Parkhill et al. 2001). Moreover, the pseudogenes in S. Typhi are not spread randomly throughout the genome: they are overrepresented in genes that are Salmonella specific when S. Typhi is compared with E. coli (59% of the pseudogenes lie in the unique regions, compared with 33% of all S. Typhi genes being unique). Many of the pseudogenes in S. Typhi have intact counterparts in S. Typhimurium that are involved in virulence and host interaction (Parkhill et al. 2001). IS26‐mediated deletions of genes in the fljAB operon prevent phase switching and therefore result in the S. Typhimurium monophasic phenotype (Laorden et al. 2010; Boland et al. 2015). Rapid, continuing flux of chromosomally encoded antimicrobial resistance genes has also been observed among closely related monophasic S. Typhimurium isolates with deletions also mediated by IS26 elements (Boland et al. 2015). Together with anthropogenic pressures, the genetic context of heavy metal resistance genes and antimicrobial resistance genes among monophasic variants may influence stability and co‐selection (Mastrorilli et al. 2018). Genes encoding resistance to mercury, copper, and silver are chromosomally encoded on Salmonella genomic island (SGI)‐4 and are almost ubiquitous in an important “European” monophasic S. Typhimurium clone (Branchu et al. 2019). The copper resistance, encoded by pco and sil operons, may have contributed to the success of this clone in pig herds, since copper has commonly been used as a growth promoter in pig production. In contrast, in other monophasic clones the pco and sil operons are plasmid‐encoded with less consistent carriage, indicative of repeated losses. IS26 elements have contributed to plasticity in the multiple drug resistance region in many variants of SGI1, which is an integrative mobilizable element with a complex integron in which antimicrobial resistance genes are clustered. A wide variety of plasmids, other than virulence‐associated plasmids, are common to multiple Salmonella serovars, many of which encode for reduced antimicrobial and/or heavy metal susceptibility. Plasmids can be associated with adaptation to host species niches, such as ColV in S. Kentucky isolates from poultry (Johnson et al. 2010). Additionally, plasmids can confer fitness benefits in particular environments such as ColE1 plasmids carried by S. Heidelberg in poultry litter (Oladeinde et al. 2018). All of the published Salmonella genomes are polylysogenic, carrying between three to five integrated bacteriophages (prophages) as well as several prophage remnants. In addition to contributing to the overall DNA diversity differentiating both Salmonella lineages and isolates, bacteriophage can also act as vectors carrying “cargo genes,” which are not essential for phage proliferation, but which can impinge directly on the pathogenic potential of their host. The phenomenon of lysogenic conversion is well described within S. enterica subsp. enterica serovars including S. Choleraesuis and S. Typhimurium, which harbors several lysogenic bacteriophage: Gifsy‐1, Gifsy‐2, Fels‐2, and SopE (Figueroa‐Bossi et al. 2001). The genetic cargo of these prophages includes the sopE gene, encoding a T3SS effector protein shown to stimulate GDP/ GTP nucleotide exchange in several Rho GTPases in vitro, and sodCI, a Cu/Zn periplasmic superoxide dismutase (SOD) that protects the host against oxidative stress. Many of the S. Typhimurium prophage cargo genes have been shown to increase the pathogenicity of S. Typhimurium in various models including mice, cattle, and macrophage (Figueroa‐Bossi et al. 2001). For example, curing S. Typhimurium strains of Gifsy‐2 results in an around 100‐fold attenuation of virulence in a mouse model of disease. Genome analysis has also shown evidence that prophages have had a long‐term role in Salmonella evolution. Several Salmonella virulence determinants such as the T3SS effector protein SspH2 and the PhoPQ‐activated genes pagKMO and various lipopolysaccharide (LPS) modification genes are surrounded by prophage gene remnants. S. enterica subsp. enterica serovars are pathogens of warm‐blooded animals, which, by their inability to ferment lactose, are considered to have been associated with intestinal colonization of reptiles and birds. Their metabolic flexibility, which incorporates a degree of redundancy, enables them to survive outside the host for periods of time and to colonize a variety of host species. The production of the variety of disease observed is associated in some cases with specific serovars. S. enterica subsp. enterica remains a human and animal pathogen of worldwide significance, and it has remained at the center of microbiology for several decades, with frequent reviews of our scientific understanding and practical significance (Neidhardt et al. 1996; Mastroeni and Maskell 2006; Barrow and Methner 2013). Based on pathogenesis and infection biology, Salmonella serovars can be classified by production of at least three distinct types of infection (pathovars). A small number of serovars are able to produce severe systemic disease in immunologically and physiologically normal, outbred, healthy adult individuals of a narrow range of animal species. Transmission is generally via the fecal–oral route, and bacterial multiplication is widely considered to take place primarily in the cells of the macrophage monocyte lineage. In the target species, the alimentary tract becomes involved pathologically only in the later stages of the disease and, in the absence of disease, little intestinal colonization takes place. Such infections are associated with S. enterica subsp. enterica serovar Typhi (S. Typhi) and Paratyphi A and some strains of Paratyphi B, which produce typhoid in humans, S. Gallinarum in poultry and probably other birds, and S. Choleraesuis in pigs. Another subgroup of serovars is associated with systemic infections; infection frequently involves the reproductive tract when animals are pregnant (mammals) or in egg lay (birds). These serovars may also be involved in enteritis and more extensive systemic multiplication in very young animals. These include S. Abortusequi in horses, S. Abortusovis in sheep, S. Dublin in cattle, and S. Pullorum in birds. Most of these serovars show persistent infection after convalescence which is an important aspect of the epidemiology. The vast majority of the remaining serovars are unable to produce systemic infections in normal, healthy adult animals. They are, however, able to colonize the alimentary tract of a range of animals and may cause acute enteritis or subclinical infections. The molecular basis of Salmonella‐induced enteropathogenesis is now better understood (see below). As a result of intestinal colonization and high levels of fecal shedding, particularly in food animals, bacteria may enter the human food chain causing gastroenteritis (food poisoning). In addition, and as members of two major pathovars, serovars S. Enteritidis and S. Typhimurium are most frequently associated with human infection and thus colonize the alimentary tract of food‐producing animals. They are also capable of producing typical typhoid infections, in this case in Slc11a1−/− mice. They are also able to produce severe systemic disease in very young animals, including poultry younger than a few days and in calves and pigs, which may have lower concentrations of protective colostrum‐derived specific immunoglobulin G (IgG) in their blood. It is interesting that other serovars, including S. Abortusovis, S. Choleraesuis and S. Dublin are also able to produce typhoid in mice. A classification of Salmonella virulence may also be made on the basis of host range, although there is disagreement over the terminology. In one school of thought, association with host species is the basis for division into three groups. Host‐specific serovars typically cause systemic disease in a limited number of phylogenetically related species, as indicated above. Thus, S. Typhi, S. Gallinarum, and S. Abortusovis are almost exclusively associated with systemic disease in humans, fowl, and sheep, respectively. Host‐restricted strains, while primarily associated with one or two closely related host species, may also infrequently cause disease in other hosts. For example, S. Dublin and S. Choleraesuis are generally associated with severe systemic disease in ruminants and pigs, respectively, but both can cause disease in calves and rodents. In the United Kingdom, 99% of all S. Choleraesuis incidents are associated with pigs and 95% of all S. Dublin incidents are associated with cattle. In contrast, the ubiquitous serovars, such as S. Typhimurium and S. Enteritidis are able to induce gastroenteritis in a broad range of unrelated host species. Another school of thought divides the serovars into the two main groups producing different infection and disease scenarios (pathovars): those that typically produce systemic disease and those that colonize the intestine and produce enteritis. In this model, the ability to produce typical typhoid‐like infections is restricted to those serovars that produce disease either in mammals (S. Dublin, S. Typhimurium, S. Enteritidis in mice, S. Choleraesuis in a wide range of mammalian species, and S. Typhi and Paratyphi, restricted to humans) or in avian species (S. Gallinarum and its related serovar, S. Pullorum). The experimental evidence tends to support this classification, with some provisos. Thus, the fact that typical typhoid may be produced in mice by selected serovars suggests an increased susceptibility in certain strains of mice. S. Choleraesuis is highly virulent and produces typhoid experimentally in mice, rats, guinea pigs, rabbits, as well as in calves. In contrast, S. Typhi and Paratyphi produce typical infection only in humans. All these serovars never produce typical infections in adult birds. The contrary is also true, that S. Gallinarum and S. Pullorum never produce typical typhoid in mammals. There appears to be no clear host species association based on ability to cause enteritis. Strains of S. Typhimurium produce enteritis in a variety of adult mammals including humans. The situation with other serovars is less clear, although a variety of serovars produce enteritis in the young of several species including cattle, pigs, sheep, companion animals, and poultry. Age has an enormous effect on disease susceptibility. Typically, very young animals are highly susceptible to Salmonella infections both as a result of a simple, noninhibitory intestinal flora which takes time to mature, and immunological immaturity. Variation in disease can be observed which shows clearly that the age of the animal at infection is important in development of disease. S. enterica infection of young chickens of less than three days old can lead to severe disease (Smith and Tucker 1980). In contrast, no clinical signs are observed with infection of healthy adult chickens (Barrow et al. 1988). This is likely to be the result of increased neutrophil/heterophil granulocyte numbers and maturity. Thus, depletion of mature chickens of heterophils using 5‐fluorouracil induces a degree of susceptibility to systemic salmonellosis normally seen only in newly hatched birds (Kogut et al. 1993). Some of the factors associated with age are poorly defined and understood. Thus, genetic differences in susceptibility to intestinal colonization of chickens by Salmonella are clear when the birds have a mature gut flora and are at least six weeks of age, but these differences are not expressed until the birds reach this age (Barrow et al. 2003; Beal et al. 2006). Except where work is referenced specifically, the following sections on the nature of the disease in domestic animal species summarizes the relevant sections in Stableforth and Galloway (1959) and Barrow and Methner (2013). Salmonellosis in cattle occurs worldwide and is associated primarily with serovars Dublin and Typhimurium. During the past 20 years, the next most frequently isolated 10 serovars other than S. Dublin and S. Typhimurium accounted for less than 10% of incidents in the United Kingdom. S. Dublin and S. Typhimurium are endemic in northern Europe, although the distributions of these serovars differ. In the United Kingdom, S. Typhimurium occurs in all geographical regions, whereas S. Dublin is predominately found in north‐ and southwestern England and Wales. In the United States, S. Typhimurium is endemic in cattle throughout the country. In contrast, S. Dublin, which before the 1980s occurred only to the west of the Rocky Mountains, has recently spread eastwards to other states and north into Canada, from where it had not previously been isolated. Salmonellosis reached a peak in the British cattle industry in the 1960s, with over 4000 incidents in 1969, most of these being associated with infections in young calves, predominantly involving S. Dublin. More recently, there has been a steep decline in the number of Salmonella outbreaks, and over the past five years there have been 400–500 incidents per annum, with approximately equal numbers of incidents caused by S. Dublin and S. Typhimurium or Montevideo. Epidemiological analysis of S. Dublin by electrophoresis indicated three phenotypes, one of which is global in distribution and in which one, in the United States, non‐motile strains are common. Vi‐producing strains were restricted to one of the taxa, isolated from the United Kingdom and France at that time. Phage typing of S. Dublin also indicates that a small number of types predominate. This, together with the fact that most infections are limited within herds may, to some extent account for the absence of multiple antibiotic resistance in S. Dublin which is frequent in S. Typhimurium. In S. Typhimurium, a small number of strains also tend to predominate but these tend to be multiresistant; PT29 in the 1960s, 204c in the 1990s, and DT104 until recently, although this is now becoming less dominant with increasing frequency of isolation of DT193 but with low isolation of the mono‐phasic variant. In calves, clinical disease is most common at two to six weeks of age. Clinical signs vary, but typically the enteric form of disease predominates which is characterized by pyrexia, dullness, and anorexia, followed by diarrhea that may contain fibrin and mucous. Pneumonia may also occur. The feces may become blood stained and “stringy” due to the presence of necrotic intestinal mucosa. Calves rapidly become weak and dehydrated, and unless treated, infected calves usually die five to seven days after the onset of disease. At this stage, the organism has become systemic, probably largely as a result of reduced innate immunity, and may be isolated from a variety of tissues, including the blood. Calves that recover from infection do not typically remain carriers. Salmonellosis is very variable and, in some animals, particularly the very young, rapid multiplication occurs both in the intestine and systemically, associated with poor absorption of specific IgG from colostrum or with calves receiving insufficient or no colostrum. This may be accompanied by true septicemia, although this has never been accurately determined, and animals may die in a comatose state after three to five days in the absence of diarrhea, as a result of high levels of systemic bacterial endotoxin. The breed of animal can also affect the outcome with some breeds, such as the Jersey, being far more susceptible than Friesian and beef breeds. In adult cattle, both acute and subacute forms of disease are recognized, caused by S. Dublin or, less frequently, S. Typhimurium or other serovars, including S. Enteritidis. The onset of the severe, acute form of disease is sudden and typically accompanied by pyrexia, dullness, anorexia, and reduced milk yield. Severe diarrhea follows, which may contain blood, mucus, and necrotic intestinal mucosa. Early in infection, Salmonella may be isolated from blood and milk in addition to the feces. Pregnant animals generally abort following S. Dublin infection. High temperatures usually persist for several days and then fall just prior to death (one to five days after infection). Abortion may occur, particularly with S. Dublin infections. Experimental work in rats has indicated that preinfection with parasites such as Fasciola hepatica, Babesia or the nematode Nippostrongylus brasiliensis increases the severity of disease. Infected cattle may excrete up to 108 colony forming units (CFU) Salmonella/gram of feces, and environmental contamination is thus a potent source of infection. Subclinical excretion of Salmonella occurs in which cattle excrete Salmonella in concentrations greater than 105 CFU/g of feces. Active carriage is usually the sequel to clinical enteritis or systemic infection, and infected animals may occasionally excrete Salmonella for years or even for life. In some animals, known as “latent carriers,” Salmonella persists subclinically in the tissues but are only intermittently excreted in feces. Excretion may be activated by stress, for example, at parturition or following parasite infection. Compared with bovine salmonellosis, the general incidence of disease in sheep in most countries is low, reflecting their generally less intensive management. In many countries, including Mediterranean countries, disease caused by the host‐adapted strain S. Abortusovis remains an important economic problem. In many other countries, it is a relatively minor problem and other serovars, such as S. Typhimurium, are predominant. S. Dublin may also produce disease in sheep. Currently, the most frequently isolated serovars are S. diarizonae (O61:k:1,5,7), S. Derby, S. Montevideo and S. Newport, but the list is by no means restricted to these. S. Montevideo has been associated with abortion in ewes for a number of years. This is caused largely by a single biotype, which is different from that causing infections in man, cattle, and poultry. In the United States, Canada, and some European countries, S. diarizonae is important. In comparison with bovine salmonellosis, there is much less information on the routes of transmission. S. Abortusovis may be isolated from the genitalia of both female and male sheep, but the extent to which transmission occurs by this route and is responsible for infection is disputed. In the case of S. diarizonae, nasal carriage also appears to be an important aspect of the epidemiology. As with S. Dublin infection in cattle, other factors may precipitate disease in carriers. These include Chlamydia infection, chemoprophylaxis, nutritional factors including drought, and other factors inducing stress, such as cold and overcrowding. The course of infection is similar with S. Abortusovis and S. Montevideo, with mortality varying between 10% and 75%. In adult animals, signs are variable and may be few prior to abortion. Profuse growth of S. Abortusovis may be obtained from embryonic tissues. This generally does not appear to affect the ewe greatly which may lamb normally in the next season and after abortion, excretion is short lived. Lambs may also be stillborn and may die soon after birth, or septicemia may occur during the first few weeks of life, although little is known of the pathogenesis of these infections. In addition, S. Montevideo may also be excreted in the feces without abortion. The serovars of Salmonella associated with clinical disease in pigs can be divided into two groups: the host‐restricted serovars typified by S. Choleraesuis and the ubiquitous serovars typified by S. Typhimurium. Since the 1960s, the occurrence of S. Choleraesuis has fallen dramatically in the United Kingdom and is now only isolated sporadically, whereas it remains a major threat to the pig industry in the United States and in Asia. In recent years, monophasic variants of S. Typhimurium, mainly 4, 12:I,‐ and 4, 5, 12:i,‐ have become increasingly important serotypes in pigs with less frequent isolation from other host species. These are monophasic variants of the predominant phage type (e.g. DT 193) and it is interesting to speculate on whether and why evolution is occurring in real time, given the occurrence and advantage in systemic disease of monophasic strains (Dublin and Enteritidis) or nonmotile strains (Gallinarum and Pullorum) elsewhere in food‐producing animals (see below). S. Derby and S. Bovismorbificans are also isolated from pigs in the UK and other countries although much less frequently than Typhimurium and the monophasic derivatives. fewer than 46% of healthy pigs in the United Kingdom can have serological evidence of Salmonella infection. Oral ingestion is thought to be an important route of infection as Salmonella are shed in high numbers in the feces of clinically infected pigs, although high doses of between 108 and 1011 CFU are required to cause disease experimentally. Pneumonia is a common feature of S. Choleraesuis infections in pigs, and several studies have shown that pigs can be experimentally infected by intranasal inoculation. The tonsils and lungs are thought to be important sites of invasion. Clinical salmonellosis in pigs generally takes two forms: septicemia caused by host‐restricted serovars such as Choleraesuis, and enterocolitis caused by broad host‐range serovars such as S. Typhimurium. Not surprisingly, weaned pigs that are intensively reared are most frequently affected by Salmonella infections. S. Choleraesuis has the capacity to cause disease in both young and older animals, whereas S. Typhimurium typically causes disease in pigs aged between 6 and 12 weeks, but rarely in adult animals. In older animals, subclinical infections with S. Typhimurium are frequent, leading to high transmission rates if active carrier animals are not detected. Pigs infected with S. Choleraesuis are lethargic, pyrexic, and often with respiratory symptoms, including coughing. Diarrhea may or may not be present, and cyanosis of the extremities is common. In most cases, mortality is high. S. Typhimurium typically causes enterocolitis with a watery diarrhea. Pigs become anorexic, lethargic, and febrile, but mortality is typically low. Pigs are frequently associated with subclinical infections caused by other serovars of Salmonella. The different forms of porcine salmonellosis can be reproduced experimentally. There is some evidence that serovars Typhimurium and Choleraesuis may invade the intestinal mucosa by distinct routes. Following oral infection, S. Typhimurium shows low invasiveness for the enteric mucosa and does not reveal any tropism for a specific intestinal location. However, S. Choleraesuis can be found in the colon and on the luminal surface of ileal M cells of Peyer’s patches. More recently, it was reported that serovars Choleraesuis and Typhimurium invaded enterocytes, goblet cells, and M cells in porcine ileal mucosa, but that Choleraesuis was found more frequently within M cells than S. Typhimurium. In addition, Choleraesuis appears to induce less damage to the mucosa than does Typhimurium, which is consistent with the hypothesis that host‐specific serovars cause systemic disease by a strategy of stealth (see below). Global poultry production has undergone many changes in the past 30 years, with the center of gravity slowly moving away from Europe and the United States to South America and Asia (although broiler and layer numbers have also increased in the United Kingdom and some other countries in the past 10 years). In several of the countries involved, there is a mix of backyard flocks and large integrated poultry production companies. In some cases, large companies subcontract production to smaller flocks, many of which will have currently limited understanding of the need for strict biosecurity; regulation of antibiotic use is also limited or nonexistent. The prevalence of Salmonella serovars in domestic fowl varies in different countries and with time, with serovars emerging and then disappearing with no obvious cause. In the 1950s, S. Agona was prevalent in UK poultry industries, yet disappeared without any obvious intervention. Historically, S. Typhimurium has been among the most prevalent serovars isolated from poultry. In the United Kingdom, between the years 1968 and 1973, S. Typhimurium accounted for over 40% of all Salmonella isolations associated with poultry, followed by S. Enteritidis (6%), S. Pullorum (4%), and S. Gallinarum (3%). During this time, S. Hadar became established in the UK poultry industry, initially in turkeys and then also in chickens. During the 1980s, S. Enteritidis phage type 4 emerged as the predominant serovar, exceeding the isolation rates of S. Typhimurium. These two serovars remain dominant although the phage type of enteritis varied between countries, with phage types 8 and 13a also common in many countries. After S. Typhimurium and S. Enteritidis, the most commonly isolated serovars are a monophasic variant of Typhimurium 1,4,[5],12:I;‐, Anatum, Infantis, Kentucky and Sofia, all important in different parts of the world. The continued high prevalence of S. Enteritidis, S. Typhimurium, and other serovars in poultry is reflected in many other parts of the world where multiresistance to antibiotics may also be a very common feature. There are some exceptions; for example, S. Enteritidis has been virtually absent from Australasia, likely the result of strict controls on importation of poultry. However, these countries have their own epidemiological peculiarities, such as the prevalence of S. enterica subsp. salamae ser. Sofia in broiler chickens. Interestingly, this is not reflected in a high incidence of S. Sofia infections in humans. The poultry‐specific typhoid serovars S. Gallinarum and S. Pullorum have largely been eradicated from the poultry industries of Europe and North America. However, in regions of the world, especially those which experience high ambient temperature and limited biosecurity, these serovars still represent major threats to bird health, welfare, and the local economy. Although chickens are the most economically important hosts for S. Gallinarum and S. Pullorum, natural outbreaks caused by these serovars have been described in turkeys, guinea fowl, and other avian species. In addition, the increase in the popularity of free‐range rearing in northern Europe has resulted in isolation of S. Pullorum infection in poultry, presumably from wild birds. The sources of infection in poultry are many, including poultry themselves, feed, and the environment and in the cases of S. Gallinarum, S. Pullorum, and S. Enteritidis, vertical transmission occurs. Fowl typhoid is widely considered to be a disease of adult birds and Pullorum disease a disease of chicks and poults; however, S. Gallinarum infects both young and older birds. Horizontal transmission of S. Gallinarum is largely fecal–oral, and the organism colonizes the gut very poorly but multiplies in the monocyte‐macrophage cell lineage in the spleen and liver. As a result of the ensuing bacteremia they localize in the intestinal wall, possibly in aggregates of lymphoid cells and are shed into the intestine. Surviving birds show large areas of sterile necrosis in the myocardium, from which, unlike the case with S. Enteritidis, it is not possible to isolate viable bacteria. Older literature, involving epidemiological studies, suggests that vertical transmission of S. Gallinarum is an important mode of spread. However, it is very difficult to reproduce this experimentally. The evolution of Gallinarum and Pullorum is complex with the suggestion that they originated in a serovar similar but not identical to Enteritidis with gene loss occurring before and after they both diverged from a common ancestor (Langridge et al. 2015; Matthews et al. 2015). However, Pullorum is less virulent in older birds than S. Gallinarum, with high mortality occurring only when birds are infected within a few days of hatching. In surviving birds, the organism persists in the spleen and despite high levels of circulating antibody, it is not eliminated for months. When the hens become sexually mature at 16–20 weeks, the organism multiplies in the tissues again and spreads to other sites, including the reproductive tract, and particularly the ovaries, from where eggs may become infected (Wigley et al. 2001). The tropism of S. Pullorum for the reproductive tract is much greater than is the case for the ubiquitous serovars. S. Pullorum readily infects the reproductive tract and developing eggs following oral infection, with particularly high numbers in the oviduct at the point of lay (Wigley et al. 2001). The molecular basis for this tissue tropism remains unknown. The capacity of serovars other than Gallinarum and Pullorum to cause disease is relatively poorly understood. S. Typhimurium, S. Enteritidis, and some strains of other serovars are capable of producing clinical salmonellosis in very young birds, probably by a mechanism similar to that of S. Pullorum. The reasons why serovars such as Heidelberg, Seftenberg, Infantis, Montevideo, and Menston are apparently much less virulent in chicks yet retain the capacity to colonize the intestine and cause human food poisoning are not understood, although these latter strains do not possess the virulence plasmid (see below). Recent studies have shown that during macrophage infection in vitro SPI‐1 genes are not downregulated, as occurs with S. Typhimurium and S. Enteritidis and it may be significant that the SpiA protein in Salmonella, truncated in some typhoid serovars including Gallinarum, has immune suppressive abilities suggesting that this may also account for the more persistent colonization of the alimentary tract in comparison with Typhimurium and Enteritidis. Mortality rates, which vary from less than 10% to more than 80%, probably result from a combination of endotoxicity and dehydration. Strains of S. Enteritidis, in addition to being highly virulent for young chicks, can cause asymptomatic and chronic infections in older birds including commercial layers and broiler breeders. Infection with this serovar soon after hatching can result in infection persisting until birds come into lay followed by vertical transmission. The extent to which egg contamination is a result of systemic bacteria reaching ovules, or a result of contamination, either in the oviduct or cloacae after egg formation and the extent to which this is a characteristic unique to S. Enteritidis, remain unclear. The inner shell and the shell membranes are frequently the main site of infection and egg infection may continue after fecal excretion ceases, suggesting that the lower reproductive tract (isthmus and uterus) are the main sites of colonization. S. Enteritidis colonization of the preovulatory follicles and ability to attach to ovarian granulosa cells has been demonstrated, suggesting that infection of eggs may occur as a result of systemic infection. S. Enteritidis may also be able to colonize the reproductive tract by virtue of its ability to bind to secretions within the isthmus using type 1 fimbriae. However, all this latter work was carried out using in vitro assays. It is thought that S. Enteritidis expresses a number of antimicrobial peptide resistance proteins which assist persistent and silent colonization. Other serovars are also occasionally able to colonize the reproductive tract and, although the basis is unknown, suppression of CD4 and CD8 lymphocytes has been proposed to be involved. Salmonella infections have been considered to have several discrete stages, although these are clearly interlinked: attachment, invasion with enteropathogenesis, and the systemic/intracellular phase. In addition to their role in intestinal colonization, which may include association with the mucosa (section above), fimbriae have been implicated in attachment and invasion. Loss of selected fimbriae in S. Typhimurium leads to reduced epithelial invasion and virulence (Bäumler et al. 1997; Van der Velden et al. 1998). In addition to adhesins, the type III secretion system‐1 (T3SS‐1) also play a role in enteric colonization suggesting some form of cycling between lumen and mucosa (Morgan et al. 2004). Invasion, the enteropathogenic response, intracellular survival and other virulence genes are tightly linked to genes encoded on SPIs. Pathogenicity islands are discrete inherited areas of the genome that vary in the numbers of genes they contain and in their role. Of the 23 SPIs described, five (SPI‐1 to SPI‐5) appear to be common to all serovars of S. enterica. The remainder are distributed among different serovars. Arguably, the two key SPIs are SPI‐1 and SPI‐2, which contain the type three secretion systems TTSS‐1 and TTSS‐2, respectively. These two islands were initially reported as being involved in invasion and intracellular survival (see section below). However, other genes and islands often encoding T3SS effector proteins are involved in invasion and intracellular survival. The T3SS promote the transfer of effector proteins from bacteria in contact with infected host cells membranes directly into the host cell cytoplasm where they have a range of effects. A number of transferred effector proteins have been characterized in a range of bacterial species. SPI‐1 is approximately 40 kb in size, has a lower GC content in comparison with the rest of the genome, and is composed of two main elements. The SPI‐1 TTSS is encoded by approximately 30 genes, whose products form needle‐like surface appendages that mediate the delivery of effector proteins into the host cell. A small second element sitABCD flanking SPI‐1 encodes an iron uptake system not thought to be related to invasion and enteritis. SPI‐1 promotes invasion in a number of Salmonella serotypes including Gallinarum, Typhimurium, Pullorum, and Enteritidis, and is also present in other serotypes which do not generally produce systemic disease but are associated with gastroenteritis, such as Hadar and Infantis (www.sanger.ac.uk/projects/microbes). A range of effector proteins are found on other pathogenicity islands associated with T3SS. While SPIs play a key role in infection, other critical factors are involved in disease. The virulence plasmids are also vital for infection to proceed and are found in most serovars associated with systemic disease (see below). Although these are known to be important in the systemic phase of the disease, their encoded fimbriae play a role in colonization and invasion, as can be seen with S. Gallinarum (Rychlik et al. 1998). TTSS‐1 is considered responsible for mediating the intestinal invasive phase of Salmonella infection. The genes are induced in vitro under conditions including late log‐phase growth in rich media containing high levels of salt and increased temperature, conditions that are thought to replicate entry into the environment of the small intestine. T3SS‐1 genes are also expressed on contact with host cells. Following attachment, Sip proteins (Salmonella inner proteins) are translocated into the cell and SipC localizes with the cell membrane to facilitate the transfer of further Salmonella effectors. Subsequent to this, at least 20 other effectors are injected into host cells. SipA interacts with SipC to block actin depolymerization factor, stabilizing F‐actin filaments at the site of bacterial attachment (Hayward and Koronakis 1999; Jepson et al. 2001; McGhie et al. 2001). The effector proteins SopE and SopE2, guanine exchange factors, then promote filopodia extensions or membrane ruffles (Bakshi et al. 2000; Stender et al. 2000). While SopB modifies phosphoinositide metabolism and indirectly activates Rho GTPase leading to micropinocytosis through activation of Wiskott–Aldrich syndrome protein (Hernandez et al. 2004). Together, these processes promote bacterial envelopment. The effector SptC has an essential role in antagonizing the function of the SopE/E2 effectors, allowing for recovery of cellular architecture post invasion (Fu and Galan 1999; Stebbins and Galán 2000; Kubori and Galan 2003
8
Salmonella
Introduction
Characteristics of the Organism
Classification
The Genome of Salmonella enterica
Serovar
Typhimurium
Typhi
Choleraesuis
Paratyphi A
Enteritidis
Gallinarum
Strain
LT2
CT18
SC‐B67
SARB42
P125109
287/91
Size (bps)
4 857 432
4 809 037
4 755 700
4 585 229
4 685 848
4 658 697
% G + C
52.22
52.09
52.11
53
52.17
52.2
CDS (n)
4451
4599
4445
4263
4318
4274
Coding density (%)
86.80
87.60
83.90
82.50
85.50
79.90
Average gene size (bps)
947
958
898
924
953
939
rRNA operons (n)
7
7
7
7
7
7
tRNA (n)
85
78
85
82
84
75
Pseudogenes (n)
25
204
151
173
113
309
Plasmids (n)
1
2
2
0
2
2
Variation in the Genome of Different Serovars
Pangenome, Horizontal Genetic Transfer and Gene Loss
Pathogenicity Islands
Gene Rearrangements
Plasmids and Prophages
Types of Disease and Pathologic Changes
Salmonella Pathovars
Salmonella Infections in Different Major Farmed Animal Species
Salmonella Infections of Cattle
Salmonellosis in Calves
Salmonellosis in Adult Cattle
Salmonella Infections of Sheep
Salmonella Infections of Pigs
Infections of Domestic Fowl and Other Avian Species
Virulence Factors
Attachment
Invasion and Enteropathogenic Response
SPI‐1 and Invasion
Stay updated, free articles. Join our Telegram channel
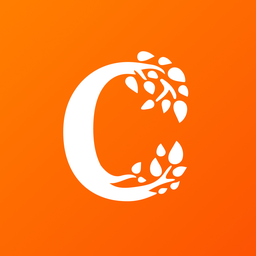
Full access? Get Clinical Tree
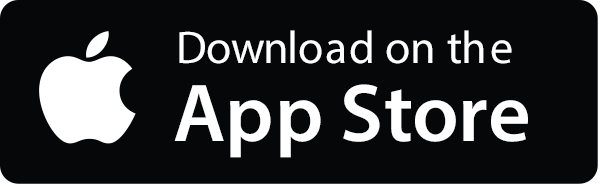
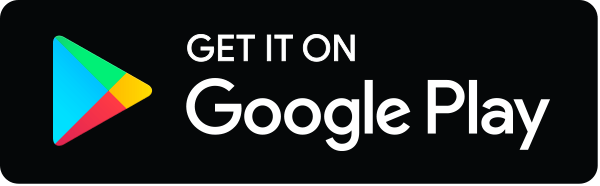