Jere W. McBride Roman R. Ganta and David H. Walker Members of the order Rickettsiales of the class Alphaproteobacteria are Gram‐negative, obligately intracellular bacteria transmitted primarily by arthropod vectors. They cause many important life‐threatening infections in livestock and companion animals and emerging zoonoses in humans. The order Rickettsiales is composed of two families, Anaplasmataceae and Rickettsiaceae. These families have major genera Anaplasma, Ehrlichia and Neorickettsia (Anaplasmataceae) and Rickettsia (Rickettsiaceae) that contain multiple pathogens of importance to veterinary medicine (Table 21.1). While Ehrlichia, Anaplasma and Rickettsia species are primarily tick transmitted, Neorickettsia species are transmitted by ingestion of infected trematodes. In the 2000s, new rickettsial pathogens of veterinary importance that have been identified include Ehrlichia minasensis, Neorickettsia findlayensis, and Ehrlichia japonica. Organisms in the order Rickettsiales are globally distributed, coinciding with presence of their arthropod vectors and natural mammalian hosts. Expansion of tick vectors and natural animal hosts of many rickettsial pathogens has been well documented and is a major contributing factor to the increasing emergence of rickettsial zoonoses in humans. Rickettsiales have evolved mechanisms to reprogram the host cell and evade innate immune defenses, and in some cases enabling persistent infection of natural hosts to maintain an infection cycle in nature. In the most recent decade, genome sequencing of many Rickettsiales have contributed to improved classification and understanding of these organisms by defining core genomes and genetic similarities and differences that may explain differential pathogenesis and host tropism (Dunning et al. 2006; Lin et al. 2017, 2021). Notably, these organisms have small genomes but have evolved strategies to establish intracellular infection of immune phagocytes, in many cases, and infect both arthropod, mammalian, and other hosts. Although substantial progress has been made in understanding the molecular and cellular pathobiology of infection, and protective antigens and immune mechanisms of these interesting intracellular microbes, there are still significant gaps in understanding the important mechanisms involved in infection and immunity. Although there has been a longstanding need for veterinary and human vaccines for diseases caused by rickettsial pathogens, this is an area that needs further attention to prevent and treat these life‐threatening infections in animals and humans. This chapter summarizes the current knowledge of major rickettsial pathogens (Table 21.1) and associated diseases in livestock and companion animals, emphasizing disease pathogenesis, immunity, and immunopathology. Table 21.1 Important rickettsial pathogens of domestic and wild animals. Anaplasmataceae pathogens are known to cause severe diseases in animals, which can also be potentially fatal (Table 21.1). Further, most of these infections may progress to a chronic stage with the pathogens persisting for long periods of time. They do not grow in standard bacteriological media and require the support of host cells for in vitro growth. Specifically, in vitro growth requires the close association of eukaryotic cells such as phagocytes or epithelial cells originating from a vertebrate or tick host. Anaplasmataceae pathogens replicate by binary fission within the phagosome of an infected eukaryotic cell, where they require host nutrient support (Pruneau 2014). This section focuses on the description of pathogenic bacteria of the genus Anaplasma impacting diverse vertebrate hosts, such as domestic and wild ruminants, dogs, cats, horses, and humans (Atif et al. 2021; Stuen 2013; André 2018; Harvey et al. 1978). Several Anaplasma species are known to be transmitted by hard ticks and cause diseases in diverse animal hosts (Table 21.1). Anaplasma marginale and A. centrale are primarily pathogens of cattle with cell tropism to erythrocytes and are causal agents of bovine anaplasmosis. Anaplasma ovis is the causative agent of ovine anaplasmosis and also infects erythrocytes (André 2018). Anaplasma phagocytophilum infects primarily granulocytes in several vertebrates, including cattle, horses, dogs, cats, and humans (Stuen et al. 2013). The disease caused by A. phagocytophilum is referred to by names associated with different host species, such as equine anaplasmosis, bovine granulocytic anaplasmosis, and human granulocytic anaplasmosis. Anaplasma platys is the canine pathogen responsible for causing cyclic thrombocytopenia because of its cell tropism for thrombocytes (platelets) (Atif et al. 2021; De Tommasi et al. 2014). Occasionally, A. platys causes infections in humans and other vertebrates. All known pathogenic Anaplasma species are transmitted by ixodid hard ticks (Hajdusek et al. 2013). Bovine anaplasmosis resulting from infection with A. marginale is a major economically important disease of cattle in the United States and many parts of the world (Aubry and Geale 2011). Economic losses arising from bovine anaplasmosis primarily result from mortality as well as morbidity of animals. Losses are estimated to be over $400 million annually for the US cattle industry alone and several billion dollars worldwide. The pathogen is maintained in infected hosts, which also act as the reservoirs of infection. Bovine anaplasmosis causes diverse clinical manifestations, including persistent high fever, anemia, icterus, weight loss, abortion in pregnant cows, lowered milk production, lethargy, and death (Kocan et al. 2003, 2010). While up to 20 different tick species have been implicated as vectors of A. marginale, it is also mechanically transmitted to naïve animals during routine management procedures, such as the use of hypodermic needle or instruments used in tattooing or dehorning (Kocan et al. 2003). Notable among tick vectors are Dermacentor species in North America and Rhipicephalus species in the rest of the world. Transmission from biting flies is another major mode of transmission of the pathogen from infected animals to naïve animals. A. marginale persists in animals recovering from the acute disease. A. marginale organisms appear within bovine erythrocytes in marginal inclusion bodies. While the bacterium cannot be cultured in standard bacterial culture media, in vitro cultivation is accomplished by serial passaging of the organism within Ixodes scapularis tick cell cultures under aerobic conditions. Anaplasma phagocytophilum infects a wide range of hosts including horses, cattle, humans, dogs, and cats (Atif et al. 2021; Stuen et al. 2013). Infections are primarily reported in the United States, Europe, and parts of East Asia. A. phagocytophilum is transmitted by I. scapularis and Ixodes pacificus in North America, Ixodes ricinus in Europe, and Ixodes persulcatus in eastern Europe and East Asia (Jaarsma et al. 2019), and the white footed mouse (Peromyscus leucopus) has been identified as the primary vertebrate reservoir (Levin et al. 2002). The pathogen infects neutrophils where it replicates within a phagosome of infected cell cytoplasm. The infectious phagosome containing the organism is referred as a morula, which may contain several replicating or infectious forms of A. phagocytophilum. Anaplasmosis in animals is typically documented in summer months when ticks are more prevalent. Anaplasma platys primarily infects canines, while occasionally, infections have been documented in humans (South America) and in cats, goats, cattle, Bactrian camels, red deer, and sika deer. A. platys infects platelets (thrombocytes) and causes cyclical changes in the platelet numbers over time as the infection progresses (Harvey et al. 1978; De Tommasi et al. 2014; Arraga‐Alvarado 2003). Thus, A. platys infection in dogs is referred as cyclic thrombocytopenia. Infections are documented globally and the tick vector for A. platys is likely the ubiquitous brown dog tick, Rhipicephalus sanguineous (Snellgrove et al. 2020). Microscopic diagnosis of infections is sometimes possible using thick blood smears stained with polychromatic stains, particularly during the clinical phases of infection. Pathogenicity of Anaplasma species is not well defined and may be complex because they cause infections in widely different host specificities and host cell associations. The pathogens may trigger immune activation leading to hypersensitivity reactions. Anaplasma species mostly infect blood cells, erythrocytes, neutrophils, or platelets, and severely impair host defense mechanisms (Rikihisa 2011; Munderloh et al. 2004; Brown 2012). The pathogens can cause high mortality rates which vary from species to species. While clinical presentation and detection of organisms in specific host cell types are frequently used as means of diagnosis, serological and molecular methods maybe useful in defining the specific infection status. Although culture isolation of an organism is possible for some bacteria, it is not a commonly used method of diagnosing Anaplasma infections, as it is time consuming, and the success of recovering an organism by cell culture isolation is very low. Cell culture methods for propagating A. platys are currently not available. The infectious form of A. marginale enters erythrocytes by endocytosis through cell surface‐expressed proteins and replicates within a phagosome by binary fission. Infectious organisms are released from infected erythrocytes by lysing the cells. Rapid loss of red blood cells occurs, and in severe cases, the erythrocyte reduction can be up to 50%. Clinical signs of bovine anaplasmosis are primarily related to the rapid loss of red blood cells and include anemia, fever, increased heartbeat, anorexia, depression, constipation, abortion, muscle weakness, myocardial hypoxia, diarrhea (Aubry and Geale 2011). During severe clinical disease, animals may exhibit spikes of fever, lethargy, inappetence, pale mucous membranes, anemia, and jaundice, and may progress to a fatal outcome. The clinical disease spectrum varies greatly depending on the age of animals and strain of the pathogen. Typically, infected calves less than six months old display few or no clinical signs, while six‐month to three‐year‐old animals may develop serious illness. Infections in cattle aged three years or more are most likely to result in mortalities up to 30–50%. Younger cattle recovering from the clinical disease may appear healthy; however, the infection persists for a long period of time, possibly for life. Chronically infected cattle with no apparent signs of illness serve as the reservoirs of infection and contribute to disease spread via tick transmission and by mechanical routes. Cattle also can be infected with the less pathogenic A. centrale. Cattle infected with A. centrale generate an immune response which appears to provide sufficient protection against the more severe clinical disease caused by A. marginale (Abdala et al. 1990). In consideration of this knowledge, infection with A. centrale followed by treatment with tetracycline derivatives is often used as a method of reducing the disease severity resulting from A. marginale infections. This method is typically employed in parts of sub‐Saharan Africa (Turton et al. 1998). The most rapid method to detect A. marginale inclusions in red blood cells of infected animals during the clinical phase of infection is microscopic examination of blood smear preparations stained with polychromatic stains (Noaman and Shayan 2010). Animals also tend to have severe anemia. Immune response in cattle against A. marginale is used as a method to diagnose the disease using an enzyme‐linked immunosorbent assay (ELISA) assay that detects antibodies to the major surface protein (MSP) 5. Molecular techniques such as polymerase chain reaction (PCR) targeting 16S rRNA gene or MSP3 gene‐specific primers are typically used to detect the infections, and are the most sensitive methods for identifying and quantifying A. marginale infection (Noaman and Shayan 2010). A. phagocytophilum infections were originally documented in ruminants in Europe with the disease commonly referred as tick‐borne fever. Subsequently, infections were documented more frequently in horses from North America, where the disease was referred to as equine ehrlichiosis caused by Ehrlichia equi (Woldehiwet 2010), later reclassified as A. phagocytophilum, the granulocytic anaplasmosis agent (Woldehiwet 2010). A. phagocytophilum infections were also reported in humans in the early 1990s, but the pathogen also infects dogs and cats. Infections with A. phagocytophilum in all hosts are known to cause thrombocytopenia, edema, and hemorrhage (El Hamiani et al. 2021). Vascular changes are more noticeable in the testes and ovaries of ruminants. Clinical symptoms may also include fever, depression, increased heart rate, decline of appetite, anemia, icterus, and ataxia (El Hamiani et al. 2021), with ataxia more common in horses. Infection‐associated neutropenia causes infected animals to become more susceptible to secondary infections (Rejmanek et al. 2012). The host immune response against A. phagocytophilum includes cellular and humoral responses. Antigenic variation in A. phagocytophilum may contribute to the persistent survival of the pathogen in an infected host by enabling immune evasion (Rejmanek et al. 2012). Significant diversity in the expressed MSPs is also reported for A. phagocytophilum strains. Recent studies suggest the translocation of the bacterial effector proteins into host cell cytoplasm and nucleus, which alters the host response and possibly extend the life span of neutrophils, both of which facilitate the bacterial replication within the phagosomes (Sinclair et al. 2015; Niu et al. 2010). Inclusions in the cytoplasm (replicating organisms in phagosomes) of granulocytes may be detected during the early clinical phase of infection following polychromatic staining of a thick smear of blood or a buffy coat sample. Indirect immunofluorescent antibody and ELISA tests have been developed. Recombinant antigens are typically used for ELISA. Molecular methods, such as PCR, are used to detect the pathogens during the clinical phase of the disease. A. phagocytophilum infection gross pathology includes enlarged spleen and localized hemorrhages (El Hamiani et al. 2021; Schäfer and Kohn 2020). Pathologic changes caused by A. phagocytophilum infections include perivascular lymphohistiocytic inflammatory infiltrates in multiple organs and hyperplasia of spleen and lymph nodes (Nair et al. 2016). A. phagocytophilum infection in dogs causes only mild inflammatory changes in lungs with scattered poorly delineated or discrete microgranulomas (Choi et al. 2007). Similarly, mild periportal inflammatory lesions occur in the liver (El Hamiani et al. 2021; Dumler et al. 2005). A. phagocytophilum and A. marginale have small genomes of about 1.2 and 1.5 Mbp, respectively, as a result of reductive evolution (Dunning et al. 2006; Foley et al. 2009; Dall’Agnol et al. 2021). The genomes have varying numbers of coding sequences in different strains which range from 890–1200 for A. marginale and 1280–1997 for A. phagocytophilum. The genomes lack genes to synthesize all amino acids, but encode genes to synthesize all major vitamins, cofactors, and nucleotides. The genomes possess many pseudogenes representing MSP superfamilies (p44 and MSP2), which appear to contribute to the bacterial antigenic variation (Palmer et al. 2000; Brayton et al. 2002; Sarkar et al. 2008). Anaplasma species have a type IV secretion system (T4SS), which is considered essential for virulence, translocates effector proteins such as ankyrin repeat domain‐containing protein A (AnkA) into the neutrophil cytoplasm (Sinclair et al. 2015; Niu et al. 2010). These effectors enable the pathogen to evade innate immune defenses and host clearance. Anaplasma species do not have genes that code for pathways for lipopolysaccharide and peptidoglycan synthesis. Anaplasma species have two morphologically distinct forms; a small nearly coccoid infectious form referred to as dense core cell (DC) and a pleomorphic form regarded as the reticulate cell (RC). The RC form resides primarily within a phagosome of a host cell, while the DC form may be present within a phagosome or extracellularly prior to infecting naïve host cells. Anaplasma species (A. phagocytophilum and A. marginale) have surface‐expressed proteins, such as the outer membrane protein A (OmpA), which function as adhesion molecules interacting with sialylated glycoproteins to enter a host cell (Ojogun et al. 2012). Additionally, AipA, Asp14, MSP4, and HSP 70 proteins in A. phagocytophilum likely contribute to the bacterial entry into a neutrophil (Contreras et al. 2017; Seidman et al. 2014; Green et al. 2010). Both A. phagocytophilum and A. marginale within a phagosome interact with host‐cell endoplasmic reticulum in both vertebrate and invertebrate host cells (endothelial, myeloid, and tick cells; Truchan et al. 2016). Endoplasmic reticulum lumen and membrane proteins, calreticulin, protein disulfide isomerase, and derlin‐1, are recruited to the periphery of phagosomes to support bacterial growth. These interactions begin at the early stages of infection and continue throughout the bacterial growth. Small ubiquitin‐like modifier (SUMO) family of proteins is essential for post‐translational modification in eukaryotes (Han et al. 2018). Altering the SUMOylation has been identified as a strategy bacterial pathogens use in support of their growth (Wilson 2012). Pathogenic bacteria, including Anaplasma species accomplish this, in part, with translocated effector proteins that negatively regulate SUMOylation. A. phagocytophilum effector protein AmpA is translocated onto the phagosomal membrane and is SUMOylated in a lysine‐dependent manner to assist in bacterial replication (Hebert et al. 2017). A. phagocytophilum secretes effector AnkA through the T4SS into infected neutrophil cytoplasm where it translocates to the nucleus and alters host gene expression to support the bacterial replication (Sinclair et al. 2015; Dumler et al. 2016). Anaplasma species require nutrient support from host cells for their efficient growth. For example, A. phagocytophilum lacks a cholesterol biosynthesis pathway. Thus, it uses the neutrophil Niemann–Pick type C1 vesicles and lipid‐raft protein flotillin of the host cell to acquire cholesterol for its continued growth (Huang et al. 2021). Similarly, the pathogen uses host‐cell autophagic machinery to acquire nutrients (Figure 21.1). This is accomplished by secreting a BECN1‐binding molecule, Ats‐1, through its T4SS, as BECN1 is needed for autophagy formation (Niu 2013). An overview of the pathogenesis of Anaplasma infections is shown in Figure 21.1. Anaplasma infections induce innate, humoral and cell‐mediated responses in vertebrate hosts. Animals infected with A. marginale develop severe anemia due to loss of erythrocytes, resulting in the decline of packed cell volume by as much as 50% within few weeks after infection. Infected younger animals may recover from the severe anemia, while the disease may progress to a fatal outcome in older animals. The pathogen can persist in animals that have recovered from the acute clinical disease. A. marginale infection elicits both humoral and cell‐mediated immune responses. Several MSPs are expressed on the pathogen cell membrane during its growth in erythrocytes (Sarkar et al. 2008; Contreras et al. 2017; Lin et al. 2004). Several, particularly MSP2, are known to have variable protein sequences, thereby contributing to antigenic variation (Sarkar et al. 2008). The MSP2 antigenic variation is identified as critical for evasion of host immune responses, thus allowing the pathogen to persist for a long period in infected hosts (Brown et al. 1998; Palmer et al. 2016). The neutrophil‐tropic A. phagocytophilum causes generalized immune suppression leading to severe leukopenia due to early lymphocytopenia, prolonged neutropenia and thrombocytopenia (Johns et al. 2009). Anaplasma infection results in decreased CD4+ T cells leading to reduced adaptive immune responses during infection (Han et al. 2008). While interferon γ (IFN‐γ) expression is regarded as important for controlling infection and pathology, it is not sufficient for complete clearance of the bacteria from a host (Bussmeyer et al. 2010). Likewise, Anaplasma infections trigger enhanced antibody production targeting several MSPs, which alone is not sufficient for pathogen clearance. Persistent infection of the host by Anaplasma is accomplished primarily via antigenic variation of immunodominant proteins of the MSP gene superfamily, such as MSP2/p44 (Palmer et al. 2016). Anaplasma genomes contain a variable number of functional pseudogenes, which are used in reorganizing the expressed gene having continuously modified hypervariable regions by gene conversion of whole pseudogenes and small segments of pseudogenes (Dall’Agnol et al. 2021). Although considerable research has been carried out to understand the variation in some of the outer surface‐expressed antigens of A. marginale and A. phagocytophilum, little is known about the pathogenesis of A. platys in a host. Antigenic variation is most likely to contribute to the cyclic thrombocytopenia in dogs infected with A. platys. Figure 21.1 Anaplasma phagocytophilum interplay with the autophagic pathway (1). A. phagocytophilum selectively recruits Rab GTPases Rab4A, Rab10, Rab11A, Rab14, Rab22A, Rab35, which regulate endocytic recycling, and Rab1, which regulates vesicular protein transport from the endoplasmic reticulum to the Golgi compartment (2). Type IV secretion system effector Ats‐1 is translocated from the ApV into the host cell cytoplasm and (3) directly interacts with autophagosome initiation complex (Atg14‐Beclin 1‐Vps34) to initiate omegasome formation in the endoplasmic reticulum (4). Isolation membrane elongates and (5)] double‐membrane autophagosome decorated with LC3 form (6)]. Autophagosomes are recruited to the ApV that fuse to release autophagic body content (7)]. A. phagocytopilum blocks lysosomal fusion potentially by preventing endosomal maturation and/or through other unknown mechanisms. Tetracycline derivatives, such as chlortetracycline and oxytetracycline, are supplemented as food additives and by the intravenous route, respectively, to control bovine anaplasmosis (Curtis et al. 2021). Enrofloxacin is another drug of choice for controlling bovine anaplasmosis (Facury‐Filho et al. 2012). However, antibiotics do not eliminate the pathogen completely from an infected animal (Curtis et al. 2021). Bovine anaplasmosis is endemic in many parts of the world, and eradicating the disease is particularly challenging due to lack of effective vaccines. Tetracycline derivatives are also the most effective antibiotics against A. phagocytophilum in treating the clinical disease. While vaccines are not available to prevent A. phagocytophilum infections in animals or humans, one of the most effective means of reducing all tick‐borne rickettsial infections, including A. phagocytophilum and A. marginale, is by controlling the exposure to ticks. Rapid advances in defining the pathogenesis of Anaplasma require the availability of molecular genetics and axenic media growth methods for these important obligately intracellular bacteria. Much remains to be understood about A. platys, and research is severely limited due to lack of in vitro culture methods for this organism. Despite considerable understanding of immunity against Anaplasma species, the molecular basis for the bacterial persistence and dual host lifecycles remains elusive. Currently, most Anaplasma infections are treated with tetracycline derivatives (Maurin et al. 2003), thus considering the resistance to clearing the pathogens with this class of drugs, it is important to develop alternative therapeutics. Similarly, research must be focused in developing effective prevention methods, such as the development of vaccines to control economically important bovine anaplasmosis and granulocytic anaplasmosis in diverse vertebrate hosts. Ehrlichia species are tick‐transmitted, obligately intracellular bacteria, and are etiologic agents long associated with important veterinary diseases such as canine monocytic ehrlichiosis (CME) and heartwater. More recently, several Ehrlichia species have also been identified as the cause of emerging life‐threatening human zoonoses, such as human monocytotropic ehrlichiosis (HME) and human ewingii ehrlichiosis (HEE; Ismail and McBride 2017). Reclassification of the Ehrlichia genus based on molecular phylogenetic analysis has identified seven taxonomically classified species: Ehrlichia canis, Ehrlichia chaffeensis, Ehrlichia ewingii, Ehrlichia ruminantium, Ehrlichia muris, with Ehrlichia minasensis, and Ehrlichia japonica (previously Ixodes ovatus ehrlichia), added most recently (Table 21.1). The first description of Rickettsia ruminantium as the cause of a febrile disease in goats, sheep and cattle was reported in 1925, by E.V. Cowdry, who described an “ultra‐visible virus” transmitted by the bont tick, Amblyomma hebraeum, which causes a disease called “heartwater.” This describes the accumulation of fluid in the pericardium that occurs during infection. The etiologic agent was later renamed Cowdria ruminantium, and more recently (2001), after phylogenetic analysis to E. ruminantium. Shortly thereafter, Rickettsia canis was identified in Algerian dogs in 1935 and was later renamed E. canis. Ehrlichial diseases gained more notoriety and attention in 1963, when E. canis was reported in the United States by Sidney Ewing and was suspected (1969) and later (1971) identified as the etiologic agent of canine tropical pancytopenia, a devastating disease responsible for deaths of a large number of US military dogs stationed in southeast Asia (Huxsoll et al. 1970). These reports established E. canis as an important canine pathogen that is known to cause CME worldwide. Near the end of the twentieth century, a novel agent, E. chaffeensis, was identified in humans in the United States. Since that time, multiple emerging ehrlichial pathogens have been associated with veterinary and zoonotic human infections, including E. ewingii (1992, dogs; 1999, humans) and E. muris subsp. eauclairensis (2009), initially referred to as E. muris‐like agent. In the past 20 years, the genome sequences of E. chaffeensis, E. canis, E. ruminantium, E. muris, E. minasensis, and E. japonica have been completed, molecular phylogenetic studies have realigned the members of the genus, immunomolecular analysis has identified important protective antigens and epitopes, protective immune mechanisms have been defined, and novel molecular pathogen–host interactions have been identified that have provided insight into the pathobiology and pathogenesis of the ehrlichioses. Ehrlichia species are tick‐transmitted pathogens, and the tick vectors include Amblyomma, Dermacentor, Ixodes, and Rhipicephalus spp. For Ehrlichia spp. that infect canines, E. canis is the most globally distributed organism, and it is transmitted by the ubiquitous brown dog tick, Rhipicephalus sanguineus. Amblyomma americanum (lone star tick) is the primary vector of E. chaffeensis and E. ewingii, which cause canine ehrlichioses and the human zoonoses, HME, and HEE. The primary Ehrlichia spp. that infects ruminants is E. ruminantium, which is transmitted by multiple Amblyomma tick species; however, A. hebraeum and A. variegatum are the primary vectors (Camus and Barre 1992). The vector for the recently identified cattle pathogen, E. minasensis, appears to be Rhipicephalus microplus, but other ticks appear to be involved in transmission in North America. Ehrlichia muris subsp. muris is transmitted by Haemaphysalis flava, and E. japonica isolated from wild mice in Japan by Hiromi Fujita, is transmitted by Ixodes ovatus (Lin et al. 2021). E. muris has been detected in other Ixodid tick species (I. cookii, I. persulcatus, and I. ricinus), and E. japonica has also been detected in I. ricinus (France and Serbia) and I. apronophorus ticks (Romania). E. muris subsp. eauclairensis is a newly identified human pathogen in the United States, where it is transmitted by I. scapularis. There have been reports that other ticks may serve as vectors of Ehrlichia spp., including H. longicornis, A. testudinarium, I. ricinus and I. persulcatus found on continents outside North America. Moreover, reports of multiple Ehrlichia spp. in R. sanguineus ticks in Africa and other locations suggest that a variety of ticks play a role in transmission depending on the geographic location. Ehrlichia spp. are maintained in nature through persistent infection of vertebrate reservoirs (Paddock and Childs 2003). These pathogens are not transovarially transmitted but are maintained in a transstadial cycle in ticks. Ehrlichia spp. infect larvae and nymphs that feed on infected animals, and the infection progresses from one lifecycle stage to another (Paddock and Childs 2003). Adults are known to maintain infection for months and can overwinter and transmit the infection the following spring. Ehrlichia spp. naturally infect animals and cause opportunistic infections in humans (Walker and Dumler 1997). E. canis primarily infects dogs and is the etiologic agent of CME; however, some evidence of human infection by E. canis has been reported in Central and South America (Perez et al. 1996). E. canis infections have been well documented globally in regions where R. sanguineus is present. E. chaffeensis has several animal reservoirs, including white‐tailed deer and canids, but also causes life‐threatening human infections in the United States and is the etiologic agent of HME (Paddock and Childs 2003). Evidence of E. chaffeensis infection has been documented outside the United States, including Africa and Mexico. E. ewingii also naturally infects canids and white‐tailed deer, and infections, particularly in immunocompromised human patients, have been reported. E. ruminantium infects domestic and wild ruminants in sub‐Saharan Africa and is the etiologic agent of the disease known as heartwater (Allsop 2010). E. ruminantium is also found on three Caribbean islands, and an organism referred to as Panola Mountain Ehrlichia species appears to be phylogenetically most closely related to E. ruminantium. Panola Mountain Ehrlichia has been identified in Gulf Coast ticks (A. maculatum) and in ruminants on the US mainland. It causes mild disease in goats and dogs, but it does not appear to cause disease consistent with heartwater. E. minasensis, which is most closely related to E. canis, was reported in Brazil, but has also been detected in cattle from Canada (Aguiar et al. 2014). E. minasensis primarily infects cattle but has also been reported in horses and dogs. Evasion of the host immune response and persistent infection of animal hosts is a hallmark of infection by Ehrlichia spp. In dogs, the clinical course of E. canis infection has been well documented consisting of acute (one to four weeks), subclinical (months to years) and chronic phases (Waner et al. 1997). The acute phase is characterized by fever, oculonasal discharge, anorexia, depression, petechiae, lymphadenopathy, and splenomegaly. Laboratory abnormalities typically include thrombocytopenia, leukopenia and anemia. Left untreated, acute infections can progress to a subclinical phase where clinical signs are absent, but laboratory abnormalities such as thrombocytopenia are detectable. During the subclinical phase the infection can persist for months to years where dogs are carriers. Some dogs with subclinical infections recover spontaneously, or progress to a severe a chronic disease where clinical signs are apparent and more severe than the acute phase. Severe pancytopenia is often detected, and bone marrow hypoplasia is common. In the chronic stage, the prognosis is poor, and dogs often die from excessive bleeding or secondary infections due to pancytopenia. Clinical manifestations of E. chaffeensis infection in dogs appear to be less severe, and mild manifestations have been reported in experimentally infected dogs (Choi et al. 2007). The white‐tailed deer is a primary natural reservoir of E. chaffeensis, but clinical manifestations in deer appear to be absent or very mild, consisting only of transient fever. However, in humans, E. chaffeensis can cause life‐threatening manifestations that involve systemic multiorgan failure that appears to be related to a “toxic shock‐like” syndrome. Common laboratory abnormalities in dogs and humans are thrombocytopenia, leukopenia and elevated transaminases. Approximately 50% of HME cases require hospitalization, and when HME emerges, there is a 3% case fatality rate, but more recent data suggest that the rate has declined to 1%. In dogs naturally infected with E. ewingii, clinical manifestations include fever and lameness, with neutrophilic polyarthritis identified as a cause for lameness in some dogs. Laboratory abnormalities include thrombocytopenia, anemia, leukocytosis, monocytosis, and presence of reactive lymphocytes and neutrophils. Neurological signs have also been reported including ataxia, paresis, proprioceptive deficits, anisocoria, intention tremor and head tilt. Ehrlichia ruminantium infection manifests after a 10–21‐day incubation period and results in non‐specific signs including fever followed by clinical disease that is peracute, acute, subacute, or mild. The acute form is most common with fever and non‐specific signs such as dyspnea, tachycardia, anorexia, coughing, oculonasal discharge, and dullness followed by death. The subacute form follows a similar course but is longer, and recovery is more common. Peracute disease results in sudden death within one to two days without clinical signs. Clinical pathological changes include anemia, thrombocytopenia, neutropenia, eosinopenia, and leukocytosis (Van Amstel et al. 1988). The clinical manifestations of naturally occurring E. minasensis
21
Rickettsiales
Introduction
Pathogen
Primary animals affected
Disease
1. Family Anaplasmataceae
Anaplasma spp.
A. phagocytophilum
Equines, canines, felines; ruminants
Equine, canine, feline granulocytic anaplasmosis; tick‐borne fever
A. marginale/A. centrale
Bovines
Bovine anaplasmosis
A. platys
Canines
Canine infectious cyclic thrombocytopenia
A. ovis
Ovines and caprines
Ovine anaplasmosis
A. capra
Ovines
Unnamed
Ehrlichia spp.
E. canis
Canines
Canine monocytic ehrlichiosis
E. chaffeensis
Canines and ruminants
E. chaffeensis ehrlichiosis
E. ewingii
Canines
Canine granulocytic ehrlichiosis
E. minasensis
Bovines and equines
Unnamed
E. muris
Murines
Unnamed
E. japonica
Murines
Unnamed
E. ruminantium
Bovines, ovines, caprines, wild ruminants
Heartwater
Neorickettsia spp.
N. helminthoeca
Canines and ursines
Salmon poisoning, Elokomin fluke liver disease
N. risticii/N. findlayensis
Equines
Potomac horse fever
2. Family Rickettsiaceae
Rickettsia spp.
R. rickettsii
Canines
Rocky Mountain spotted fever
R. conorii
Canines
Mediterranean spotted fever
R. africae
Canines
Spotted fever rickettsiosis
R. prowazekii
Sciuridae
Louse‐borne typhus
R. typhi
Canines
Murine typhus
Family Anaplasmataceae
Anaplasma Species
Overview and Pathogenic Species
Source of Infection: Ecology and Epidemiology
Types of Disease and Pathologic Changes
Gross and Histopathologies
Genome Features and Pathogenomics
Pathogenesis
Immunity and Immunopathogenesis
Control
Gaps in Knowledge and Anticipated Future Directions
Ehrlichia Species
Overview and Pathogenic Species
Source of Infection: Ecology and Epidemiology
Types of Disease and Pathologic Changes
Stay updated, free articles. Join our Telegram channel
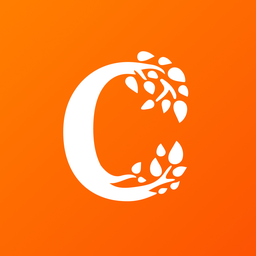
Full access? Get Clinical Tree
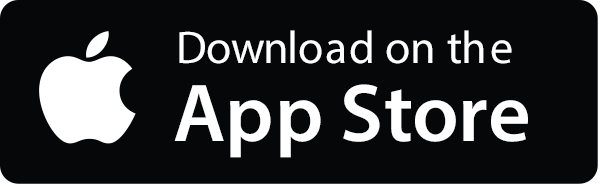
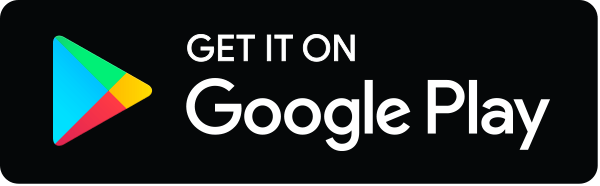