José A. Vázquez‐Boland, Macarena G. Sanz, and John F. Prescott The genus Rhodococcus is a group of metabolically versatile aerobic actinomycetes currently containing 58 defined species and an ever‐increasing number of unclassified isolates. The rhodococci are widespread in the environment and have considerable interest in industrial microbiology because of their applications in a variety of biotechnological processes, including biotransformation, biocatalysis, and bioremediation. Only two species are recognized as pathogenic, Rhodococcus equi in mammals and Rhodococcus fascians in plants. This chapter focuses on R. equi, with emphasis on recent findings. Known as a horse pathogen and opportunistic human pathogen, R. equi has recently emerged as a specialized multihost pathogen with a unique mechanism of plasmid‐driven animal host tropism, a novel paradigm in microbial pathogenesis. Rhodococci are classified in the family Nocardiaceae, order Corynebacteriales, class Actinomycetia of the Actinobacteria, also known as the high G + C Gram‐positives. They belong to the mycolata together with important genera such as Mycobacterium, Nocardia or Corynebacterium, all characterized by a protective lipid‐rich cell envelope with mycolic acids as the main constituents. R. equi are obligately aerobic, non‐motile, non‐spore‐forming, morphologically irregular coccoid to rod‐shaped bacteria. Culture in solid rich media results in heavy coalescent growth after 48 hours at 30–37°C with buff to salmon‐pigmented, smooth, glistening colonies (Figure 33.1a). Nutritionally undemanding, R. equi can grow vigorously if supplied only with ammonia, with a short‐chain organic acid as a carbon source (e.g. lactate or acetate), and thiamin for which it is auxotrophic due to a thiCD mutation (Letek et al. 2010). Named Corynebacterium equi at the time of its first description in 1923 by Magnusson in Sweden, the species was transferred to the genus Rhodococcus in 1977, but its classification remained problematic until recent phylogenomic studies unequivocally placed it within one of the major rhodococcal lines of descent (Anastasi et al. 2016). In 2014 it was renamed as Rhodococcus hoagii, but in 2022 it reverted back to R. equi by decision of the Judicial Commission of the International Committee on Systematics of Prokaryotes because the name change was proposed in error (Vázquez‐Boland et al. 2020). Like other rhodococci, R. equi is primarily an environmental bacterium. Soil is its natural habitat, but it is a coprophilic organism that multiplies in herbivore manure and in the large intestine of foals, and as a result is ubiquitous in the fecally contaminated farm environment (Takai 1997; Muscatello et al. 2007). This is aided by a number of niche‐adaptive features. A salient one is a primarily asaccharolytic metabolism, linked to absence of a phosphoenolpyrurate:carbohydrate phosphtransferase transport system (PTS), a characteristic shared with Mycobacterium tuberculosis. Primary carbon sourcing is from short‐chain organic acids and lipid catabolism. Lactate is a preferential growth substrate, with a dedicated transporter and redundant pathways for its conversion into acetate, which is also a main exogenous carbon source for R. equi. Together with a marked alkalophilia (optimal growth between pH 8.5 and 10), the metabolic profile of R. equi is likely to confer a distinct advantage in manure and the large intestine by allowing growth on byproducts of the fermentative activity of competing microbiota, such as lactate, acetate, and short‐chain fatty acids, as well as microbially derived thiamin (Letek et al. 2010). A potential capacity for denitrification via a NarK nitrate/nitrite transporter, NarGHIJ nitrate reductase and a NirBD nitrite reductase, and/or obtaining energy from H2 derived from microbial fermentative activity via a NiFe‐type hydrogenase, may help supporting R. equi growth and persistence in oxygen‐deprived habitats such as the intestine. Figure 33.1 (a) Rhodococcus equi colonies on LB medium incubated at 30°C for 48 hours. Source: Vázquez‐Boland and Meijer (2019), used under Creative Commons Attribution (CC‐BY) license. (b) Intracellular replication of GFP‐tagged R. equi in murine J774 macrophages. Source: Vázquez‐Boland et al. (2013); reproduced with kind permission of Elsevier B.V. Figure 33.2 summarizes the main metabolic characteristics and other significant traits encoded in the R. equi genome (Letek et al. 2010). The R. equi genome comprises a covalently closed circular chromosome of a little over five mega base pairs and a conjugative virulence plasmid. Two closely related circular plasmid variants of around 80–100 kb in size, designated pVAPA and pVAPB (as per a unified nomenclature for the R. equi virulence plasmids; Letek et al. 2008; Vázquez‐Boland et al. 2013), are carried by equine and porcine isolates, respectively. A third type of R. equi virulence plasmid of linear topology and around 120 kb, pVAPN (“N” standing for “No A/No B”), is present in isolates from cattle and other ruminants (Ocampo‐Sosa et al. 2007; Valero‐Rello et al. 2015; MacArthur et al. 2017). Each of the three host‐specific virulence plasmid types possesses a type‐specific copy of the horizontally acquired vap pathogenicity island, discussed under VapA Pathogenicity Island, below. The corresponding plasmid backbones share homology with other conjugative replicons found in environmental (non‐pathogenic) rhodococci, in this case carrying catabolic or detoxification pathways in their variable regions instead of virulence determinants (Figure 33.3a). Comparative genomic analyses show that R. equi is genetically homogeneous and clonal, with a large core genome equivalent to around 80% of an isolate’s gene content. All isolates radiate at relatively short genetic distances from each other, suggesting that it is an evolutionarily young species. R. equi genome evolution is primarily driven by gene gain/loss processes, with a significant contribution of horizontal gene transfer events (Letek et al. 2010; Anastasi et al. 2016). Figure 33.2 Overview of relevant metabolic and virulence‐related traits encoded in the Rhodococcus equi genome. Primarily an asaccharolytic bacterium, R. equi has been recently shown to possess RbsCB and GlcP non‐PTS sugar transporters through which it can use D‐ribose and D‐glucose, albeit inefficiently (and inconsistently for glucose; Anastasi et al. 2016). R. equi can convert lactate into acetate either directly via L‐lactate monooxygenase or via pyruvate involving a lutABC operon and pyruvate dehydrogenase (cytochrome). A putative bifunctional D‐xylulose 5‐phosphate (X5P)/fructose 6‐phosphate (F6P) phosphoketolase (Xfp) may provide flexibility in carbon and energy metabolism by catalyzing the direct conversion of the pentose phosphate pathway intermediate X5P and the glycolytic intermediate F6P into acetyl phosphate (and acetate/acetyl‐CoA). A number of mycobacterial virulence‐associated virulence gene homologs are present, including three complete mce lipid transport operons, genes encoding four Fbp/Antigen 85 mycolyltransferases, HbhA and Lbp/Hlp (laminin‐binding histone‐like protein aka HupB)‐like cytoadhesins, and MPT83 antigen‐like secreted lipoprotein (MBP70 and MBP83 in Mycobacterium bovis), as well as an esx gene cluster encoding ESAT‐6 and CFP‐10 homologs and corresponding type VII secretion system (T7SS), and a pe/ppe locus. Source: Letek et al. (2010), Public Library of Science, CC BY. R. equi infection is contracted through exposure to contaminated soil, primarily via inhalation of airborne dust particles. The exhaled breath of foals with advanced pneumonia can also be a source. Conditions favoring soil aerosolization, such as hot dry weather, high stocking density and crowded paddocks, are considered the main risk factors (Chaffin et al. 2003; Muscatello et al. 2007). On some horse farms the infection is endemic, with devastating impact. Endemic farms are generally those where foals have been intensively reared over many years, where summer temperatures are high, soil type is sandy, and dust is extensive. Dissemination of equine rhodococcosis is through movement of mares and their foals between breeding farms. Figure 33.3 (a) Comparison of the pVAPA and pVAPB circular virulence plasmids and the recently characterized ruminant‐associated linear plasmid pVAPN with corresponding closest homologs from environmental Rhodococcus spp. Regions of significant similarity are connected with gray stripes, vap pathogenicity islands (PAIs) are shaded in light blue. Green and pale red bars indicate conjugation and replication/portioning modules, dashed underline indicate horizontal gene transfer regions. (b) Genetic structure of the vap PAIs from pVAPA (15.1 kb), pVAPB (21.5 kb) and pVAPN (15.9 kb). PAI genes in gray or black (vap genes). Straight lines connect vap allelic variants, curved lines indicate vap gene duplications, crosses denote vap gene loss and asterisks, pseudogenes. (c) Fate of the vap PAI and host‐driven vap multigene family evolution. Source: Vázquez‐Boland and Meijer (2019), John Wiley & Sons, CC BY 4.0. Evidence that human infections are zoonotic has been recently provided by the identification of the animal host‐associated virulence plasmids, pVAPA, pVAPB, and pVAPN. Despite their host specificity for, respectively, equids, swine, and ruminants, each of these virulence plasmids can be found in human isolates. Among these, the porcine‐type pVAPB is the most common, followed by the ruminant‐type pVAPN and equine‐type pVAPA. A significant proportion of human isolates appear to lack a virulence plasmid, but the data before the discovery of pVAPN (Valero‐Rello et al. 2015) need to be interpreted with caution, since many strains reported as “plasmidless” turned out to be carrying the ruminant type (Ocampo‐Sosa et al. 2007; Takai et al. 2020). Phylogenomic studies provide evidence of extensive global circulation of R. equi genomotypes, presumably linked to international livestock trade (Anastasi et al. 2016). There is no obvious association between the animal host species and the chromosomal genotype; such association exists in contrast with the virulence plasmid type carried by the isolates (see Animal Species‐specific Virulence Plasmids and Host‐tropism, below). There is also evidence of active exchange of the host‐specific pVAPA, pVAPB, and pVAPN virulence plasmids across the R. equi population, accompanied by corresponding host jumps. Not only are any of the three animal host‐specific virulence plasmids commonly carried by human isolates, but strains with an identical chromosomal genomotype and host‐associated plasmid type can also be identified in both the adapted animal species and people (MacArthur et al. 2017). In ecological and evolutionary terms, humans should be considered as “occasional” or “opportunistic” hosts, as opposed to equids, swine and ruminants, which are bona fide hosts to which the bacterium is specifically adapted and from which it is transmitted to people (Vázquez‐Boland et al. 2010, 2013). Virulence plasmid loss can occur across the R. equi population independently of the genetic lineage (MacArthur et al. 2017). The proportion of R. equi strains lacking a virulence plasmid is much higher among environmental isolates compared to strains recovered from clinical specimens. About 40–50% of soil isolates (even if equine farm‐associated) do not carry a virulence plasmid, whereas virtually all freshly isolated equine clinical strains are pVAPA positive. Among strains kept in isolate collections, only 5–14% of equine origin and 0% from cattle are devoid of a virulence plasmid. Like in the case of human isolates, the virulence plasmid is absent in a significant proportion of porcine isolates (up to 30%), but these are difficult to categorize because they have mostly been collected in abattoir surveys from the tonsils of healthy carriers (in contrast to those of equine or ruminant origin, which almost invariably derive from pathological samples; Makrai et al. 2002, 2005; Ocampo‐Sosa et al. 2007; Duquesne et al. 2010). The available evidence indicates that the R. equi virulence plasmid tends to be lost in the absence of host selection, but can be easily regained from host‐associated (plasmid‐positive) bacteria (Vázquez‐Boland et al. 2013). Conjugal transfer of the R. equi virulence plasmid is efficient, with frequencies of up to 10−1/recipient (Tripathi et al. 2012). Like other related pathogenic actinomycetes, R. equi causes subacute to chronic pyogranulomatous infections, with the portal of entry defining their primary location in the body. The lungs are the organ most commonly affected. Clinical R. equi infections are relatively rare except in foals, in which they represent a significant economic burden. The most common manifestation is a life‐threatening multifocal purulent bronchopneumonia with abscessation. Exposure is thought to occur early after birth (Horowitz et al. 2001) and infection develops insidiously until onset of severe symptoms between 4 and 12 weeks of age (Giguère et al. 2011a). Clinical disease is rarely seen in adult horses even on endemic farms where a large proportion of foals manifest rhodococcal pneumonia. Progression to overwhelming pneumonia occurs in foals that do not develop an effective cell‐mediated immune response. Ulcerative typhlocolitis and mesenteric lymphadenitis may result from swallowing contaminated soil or infected sputum. Intestinal lesions are observed in approximately 50% of foals with rhodococcal pneumonia. Other less common extrapulmonary manifestations result from bacteremic spread from primary infection sites in the lungs or intestine and include osteomyelitis, purulent arthritis, and ulcerative lymphangitis. Reactive arthritis and ocular inflammatory processes may be also observed. Thoracic ultrasonographic studies have revealed that subclinical pneumonic abscesses can be very common in foals on endemically infected farms, and that most affected foals do not progress to develop clinical illness and recover without antimicrobial therapy (Venner et al. 2013). In swine and cattle, R. equi causes pyogranulomatous lesions in the submandibular and bronchial or mediastinal lymph nodes, discovered at slaughter and confused grossly with tuberculosis (TB). Carriage in pig tonsils is frequent (Makrai et al. 2005) while the prevalence in cattle may be underrecognized, as suggested by an abattoir survey in Ireland, which found R. equi in 4% of bovine TB‐like lesions (Flynn et al. 2001). Severe systemic forms with multiple caseating abscesses in the lungs, liver, and other organs have been reported in goats and camelids. Infections in other animal species, particularly dogs and cats, are rare and usually associated with immunosuppression. First isolated from a human infection in 1966, R. equi emerged as an opportunistic pathogen affecting immunocompromised people in the mid 1980s, coincident with advances in organ transplantation and cancer therapy, but particularly with the advent of the AIDS pandemic. Most cases are associated with immunosuppressive conditions and usually present as subacute cavitary pneumonia with constitutional symptoms, requiring differential diagnosis from pulmonary TB. Bacteremia and secondary dissemination are also common. R. equi infections in immunocompetent individuals are rare and tend to be localized (Weinstock and Brown 2005; Yamshchikov et al. 2010). Lesions in all species correspond to the ability of the organism to replicate in macrophages and therefore are characteristically granulomatous. Once macrophage destruction occurs through necrosis (Luhrmann et al. 2004), and in the absence of an effective type 1 cell‐mediated immune response, lesions become pyogranulomatous and progressive. The ulcerative lesions sometimes present in the Peyer’s patches of the large intestine of foals suggest an ability of the pathogen to invade cells other than those only of monocyte–macrophage origin, but this has not been proven and these alterations may result from local inflammatory or necrotizing processes. The pathological findings in humans are often described as malakoplakia, characterized by a dense infiltration of foamy histiocytes with intracellular coccobacilli and basophilic inclusions termed Michaelis–Gutmann bodies, likely representing partially destroyed intracellular bacteria (Yamshchikov et al. 2010). Resolution of lesions is the result of an effective, largely cell‐mediated, immune response, discussed in Section 33.8. R. equi infectivity is plasmid determined. Loss of the virulence plasmid renders R. equi unable to proliferate within macrophages in vitro and in mouse tissues in vivo, and to cause disease in foals (Takai et al. 1991; Hondalus and Mosser 1994; Giguère et al. 1999). The virulence plasmid is also responsible for the specific adaptation of R. equi to its three main animal hosts, i.e. equids, swine, and cattle (ruminants). These properties are linked to the vap pathogenicity island, a horizontally acquired locus inserted in the variable region of the corresponding plasmid replicons. The plasmid pathogenicity island (PAI) receives its name from the family of vap (virulence‐associated protein) genes it harbors. A total of six vap genes (plus three vap pseudogenes) are present in the 15‐kb PAI of the equine‐type plasmid pVAPA (Figure 33.3b). One of the proteins of the Vap complement encoded by each of the three host‐associated virulence plasmids, designated VapA in the equine type, VapN in the ruminant type, and VapK in the porcine type, is essential for R. equi intramacrophage replication and virulence (Jain et al. 2003; Valero‐Rello et al. 2015; Willingham‐Lane et al. 2018); the other Vaps appear to play an accessory role. The Vaps are small, secreted antigenic proteins which structurally fold in a cork‐shaped eight‐stranded antiparallel β‐barrel (Geerds et al. 2014; Whittingham et al. 2014) that provides few clues as to their mechanism of action. Based on the phenotype of a vapA mutant in infected macrophages, Vap proteins are likely to play an important role in the biogenesis of the modified phagocytic vacuole where R. equi survives and replicates intracellularly (Fernandez‐Mora et al. 2005). The vap PAI also harbors a number of conserved non‐vap genes, including the five‐gene vir operon (Figure 33.3b). Except for the virR and virS regulators in the vir operon, the precise role in virulence of the non‐vap genes of the PAI remains largely uncharacterized Plasmid virulence genes are weakly expressed at 30°C and pH 8.0, but fully activated at 37–38°C and pH 6.5 (Byrne et al. 2007). The main effect is exerted by temperature and these conditions are thought to mimic the cues sensed by R. equi during its transition from the environment to the mammalian host. Treatment with hydrogen peroxide, which reproduces the oxidative stress within activated macrophages, also results in significant increase of vap gene transcription as measured with vapA and vapG (Benoit et al. 2002). The two regulators encoded in the vir operon of the PAI, VirR and VirS (Figure 33.3b), are necessary for vap PAI virulence gene expression, but whether and how they contribute to the modulation exerted by temperature (and pH) and oxidative stress remains to be determined. Low free iron, a well‐known “host signature” cue in the control of bacterial virulence, appears to also activate the expression of vapA and the other vap genes of the pVAPA plasmid (Ren and Prescott 2003). Analysis of the virulence plasmids carried by isolates from different origins made it obvious that R. equi infectivity and specific adaptation to equids, swine and ruminants (cattle, sheep, and goats) is linked to the virulence plasmid type (pVAPA, pVAPB, and pVAPN, respectively; Ocampo‐Sosa et al. 2007; Valero‐Rello et al. 2015). The first R. equi virulence plasmid to be genomically characterized was the VapA‐encoding pVAPA, found in virulent isolates of equine origin and occasionally in human isolates (Takai et al. 2000b). Next was the VapB‐encoding pVAPB, found in isolates recovered from slaughtered pigs and human clinical specimens but never from equine isolates (Letek et al. 2008). Strains carrying pVAPB are of intermediate virulence in mice and weakly pathogenic in experimentally infected foals (Takai et al. 2000a). The more recently identified third R. equi virulence plasmid, pVAPN (Valero‐Rello et al. 2015), is found in ruminant and some human isolates but not in equine or porcine isolates (Ocampo‐Sosa et al. 2007; MacArthur et al. 2017; Suzuki et al. 2021). The equine pVAPA and porcine pVAPB plasmids are variants of the same circular replicon that differ only in vap PAI structure, specifically in vap gene complement (Letek et al. 2008). The ruminant‐associated pVAPN is an unrelated linear plasmid that carries a copy of the vap PAI (Figure 33.3a). This indicates that the R. equi vap PAI was at some point part of a mobile element, consistent with the presence of transposon invertase/resolvase or transposase/integrase genes or pseudogenes at the right flanking region of the three PAIs (Valero‐Rello et al. 2015). The vapN PAI has the same general arrangement of the vapA and vapB PAIs but again differs in a unique vap gene complement (Figure 33.3b). Exquisite conservation of the vap PAI, with 100% PAI product sequence identity (notably, of the cross‐type‐variable vap multigene family products), is observed within each host‐specific plasmid type, indicative of strong selection. Mismatches between the pVAPA/B/N plasmid type and animal host source are exceptional among equine, porcine and ruminant isolates, suggesting the existence of stringent host‐driven exclusion of the heterologous (non‐adapted) virulence plasmids (Ocampo‐Sosa et al. 2007; MacArthur et al. 2017). R. equi survival in macrophages, mice and foals requires the aceA gene encoding isocitrate lyase, a key anaplerotic enzyme essential for biosynthesis of carbohydrates and other metabolic intermediates from acetate‐ or fatty acid‐derived acetyl‐ CoA (Wall et al. 2005). Like M. tuberculosis, R. equi therefore appears to use lipids as the primary carbon source for in vivo replication, consistent with its inability to assimilate carbon from carbohydrates . Cholesterol may be critical because inactivation of ipdAB encoding a heterodimeric coenzyme A transferase in the sterol utilization pathway significantly attenuates R. equi virulence in macrophages and foals (van der Geize et al. 2011). Two other metabolic genes encoding an AroQ chorismate mutase and a bifunctional TrpE/G anthranilate synthase were shown to be required for optimal intramacrophage proliferation (Letek et al. 2010), implying that the R. equi in vivo replication niche is poor in aromatic amino acids, and/or that in vivo R. equi requires chorismate‐derived biosynthetic products such as folate, menaquinones, or siderophores. A role for the latter has been demonstrated with the hydroxamate siderophore “rhequichelin” synthesized by the R. equi non‐ribosomal peptide synthase rhbABCDE (Miranda‐Casoluengo et al. 2012; Figure 33.2). One of the four R. equi catalases, KatA (Bidaud et al. 2012), and an extracellular thioredoxin encoded by the etrx3 gene (Mourenza et al. 2020), contribute to intramacrophage survival, presumably by conferring protection against oxidative damage caused by reactive oxygen intermediates following phagocytosis. Chromosomal islands encoding pili and a capsular polysaccharide are part of the core genome of R. equi (Figure 33.2). The R. equi pili (Rpl) are of the Flp type IVb subfamily, form long appendages, and appear to promote attachment to mammalian cells (Letek et al. 2010). The importance of the R. equi exopolysaccharide in virulence is unclear because a “transposome” insertion mutant with defective capsule integrity showed no intracellular proliferation or cytotoxicity defect in macrophages and was fully virulent in mice (Sydor et al. 2008). As in M. tuberculosis, the R. equi
33
Rhodococcus equi
Introduction
Characteristics of the Organism
Niche‐Adaptive Features
Genome
Source of Infection and Epidemiology
Zoonotic Transmission
Molecular Epidemiology and Population Genomics
Types of Disease and Pathologic Changes
Foal Rhodococcosis
Disease in Other Animals
Disease in Humans
Pathologic Changes
Virulence Factors
vap Pathogenicity Island
Regulation of vap Pathogenicity Island Genes
Animal Species‐Specific Virulence Plasmids and Host Tropism
Chromosomal Virulence Determinants
Stay updated, free articles. Join our Telegram channel
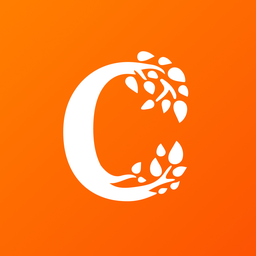
Full access? Get Clinical Tree
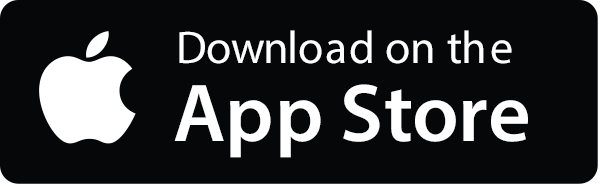
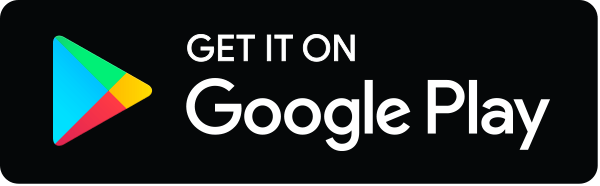