12 Radiation therapy has been used in veterinary medicine since shortly after the discovery of x-rays by Roentgen in 1895. Alois Pommer, an Austrian veterinarian, published extensively on the irradiation of benign and malignant diseases and established a radiation therapy protocol widely used for many years.1 Technologic advances improving our understanding of the radiation biology of normal and tumor tissues have enabled development of contemporary radiation therapy techniques and protocols. Over half of human patients with serious cancers undergo radiation therapy at some point during treatment.2 Radiation therapy is an effective treatment modality for animal cancer patients with solid tumors; however, early use of the modality was limited due to the sparse availability of veterinary treatment centers. The last decade has been marked by the opening of numerous veterinary radiation therapy centers and the commissioning of more advanced radiation therapy technologies. More than 60 facilities in North America are actively treating animals with radiation therapy and the American College of Veterinary Radiology (Specialty in Radiation Oncology) has residency training programs at 17 treatment centers.3 A critical determinant of a cell population’s sensitivity to radiation is the ability of cells to repair DNA damage caused by radiation. One Gy of radiation from photons causes approximately 2500 base damages, 1000 single-strand breaks, and 40 double-strand breaks in DNA in each cell.4 Most of this damage is repaired by cells within 6 to 24 hours; the double-strand breaks are the most lethal because they may lead to severe chromosomal aberrations. A given dose of radiation is preferentially cytotoxic to proliferating cells, including tumor cells and renewing cell populations (e.g., epithelial stem cells), although slowly dividing and nondividing cells (e.g., bone and cells of the nervous system) are also affected by radiation. Early radiation oncologists found that higher total doses could be given if the doses were divided into smaller fractions. They observed that tumor response was improved, and less injury of normal tissue occurred. In veterinary medicine, standard fractionation denotes a regimen delivering 2.7 to 4 Gy per fraction, 3 to 5 times per week to a total dose of 42 to 57 Gy, although several other regimens are currently being used or investigated. Hyperfractionation refers to schedules in which the dose per fraction is reduced and the total dose is increased. Accelerated fractionation describes a treatment regimen in which the overall time of treatment is reduced, but the dose per fraction and total dose are unchanged. Hypofractionation describes the administration of high doses per fraction given in a small number of fractions to a lower total dose. The response of tumor and normal tissues between fractions throughout the course of radiation therapy has been described by Withers5 as the “four Rs” of radiation therapy: repair of DNA damage, redistribution of cells in the cell cycle, reoxygenation of tumor cells, and repopulation of tumor and normal tissues. The length of time over which radiation therapy is administered is important, primarily because of tumor repopulation but also because of rapidly proliferating normal tissues, such as mucosa and skin. Tumor cells that have not been destroyed by irradiation continue to replicate during the course of therapy. This process is exacerbated by a phenomenon known as accelerated repopulation. Some have suggested that after approximately 4 weeks of therapy, tumors repopulate more rapidly than initially.6 The reason for this is not clear, but the phenomenon could be related to (1) a reduction in the cell cycle time, (2) an increase in the number of tumor cells that are actively dividing, or (3) a reduction in the number of tumor cells that normally die (cell loss factor). Regardless of the cause, when treatment lasts longer than 4 weeks, repopulation may affect the outcome unless total dose is increased to account for this phenomenon. Repopulation may have a greater impact on rapidly dividing tumors than on slowly dividing tumors. The total dose administered to a patient should have a low probability for causing significant late normal tissue reactions in the region of therapy. However, the response of tissues also depends on the fraction size. For example, 48 Gy administered in 4 Gy fractions has a higher probability of causing late effects than 48 Gy administered in 3 Gy fractions (Figure 12-1). The probability of tumor control is not as affected because rapidly proliferating tissues, including tumors, are not as sensitive to the change in dose per fraction. The benefits of protocols that use small doses per fraction are clear: they allow a higher total dose to be administered without increasing the probability of damage to late-responding normal tissues. Although time, dose and fractionation are still the underpinnings of SRT, the paradigm is different than for fractionated radiation therapy. SRT involves the use of high doses per fraction but overcomes the radiobiologic limitations with stereotactically verified positioning and treatment delivery techniques that leave a minimal volume of normal tissue in the high dose area. Stereotactic radiation therapy, by definition, requires (1) a tumor for targeting (not microscopic disease), (2) treatment planning and administration that will provide a dramatic dose drop-off between the tumor and the surrounding normal tissue structures, and (3) a method of stereotactically verifying patient positioning. The result is that normal late-responding tissue structures are spared through dose avoidance rather than by administering small doses per fraction. The normal tissue structures still receive dose, and the dose per fraction is higher than for traditional radiation therapy. However, the total dose to the normal structures is lower than what is typical for fractionated radiation therapy. Normal tissue tolerance data are just evolving for SRT, as is long-term follow-up. Estimates of tolerance have been based on limited clinical data, toxicity observation, and educated guessing.7 It is inappropriate to extrapolate dose constraints from fractionated protocols. An additional difference between SRT and fractionated radiation therapy is that with SRT, acutely responding normal tissues in the surrounding region such as skin, esophagus, and colon may be susceptible to consequential late effects. A consequential late effect is a late effect that develops from severe acute effects that may be associated with stem cell depletion. Dose constraints therefore must also be applied to these tissues. SRT treatment is generally delivered in 1 to 5 fractions over a period of 1 week or less for most tumors; therefore accelerated repopulation is unlikely to impact tumor control. Although historically stereotactic treatment was delivered in a single fraction (referred to as stereotactic radiosurgery), current technology allowing precise repositioning makes limited fractionation feasible. Even this minimal fractionation should allow higher total doses to be administered safely to late-responding tissues in the region and presumably take advantage of tumor reoxygenation and redistribution. However, biologic response following SRT has not been comprehensively evaluated. From a practical standpoint, SRT minimizes the number of anesthesia episodes to these older and sometimes debilitated patients and is also generally more convenient for the owner. Acute effects are minimal and tumor-associated signs such as discomfort or dysfunction often improve rapidly. Long-term tumor control and late effects need to be quantitated. Reactions from radiation therapy are classified as acute (also called early) or late. Acute effects occur during or shortly after radiation therapy. Acute effects involve rapidly proliferating tissues, such as the oral mucosa, intestinal epithelium, and epithelial structures of the eyes and skin. Concurrent chemotherapy can exacerbate acute effects from radiation. These effects generally are self-limiting, and recovery is rapid. However, acute effects can be unpleasant for the patient and distressing to the owner, and in rare instances they can be life-threatening if the proper care is not given. The referring veterinarian often is called on to treat recently irradiated patients. Acute effects will heal without medical intervention in the vast majority of cases over the course of weeks or occasionally months. In veterinary patients, the most important provision to allow healing during this time is prevention of self-trauma of the radiation site by the patient. Therefore pain management plays an important role and should be addressed. Pain management for cancer patients is discussed in Chapter 15, Section A, of this text, and specific protocols have been published.8 Additional treatment is based on common sense, supportive care, and the knowledge that the signs will resolve with time. Late effects involve more slowly proliferating tissues, such as bone, lung, heart, kidneys, and nervous system. The dose of radiation administered is limited by the tolerance of these normal tissue structures in the field. Late reactions can be difficult to treat; it is the radiation oncologist’s obligation to minimize the incidence of late effects with appropriate dose prescriptions and careful radiation planning and treatment. When late effects occur, they may be quite severe, resulting in fibrosis, necrosis, loss of function, or even death.9 Late effects occur from the loss of normal tissue stem cells with concurrent radiation-induced vascular changes and inflammation. These changes are multifactorial, but the cytokine transforming growth factor-β (TGF-β) is believed to play a critical role in radiation fibrosis. Strategies attempted in human radiation oncology to mitigate late radiation effects include the use of antioxidants and free radical scavengers (superoxide dismutase, vitamin E, thiol radioprotectors), vascular-directed therapies (clopidogrel, statins, pentoxifylline), antiinflammatory agents (corticosteroids), inhibitors of the renin-angiotensin system (angiotensin-converting enzyme [ACE] inhibitors), and stem cell therapies.9 In veterinary patients, severe late reactions such as fibrosis and tissue necrosis should be managed under the guidance of or referred to a surgeon and/or radiation oncologist experienced in dealing with radiation injury. Ionizing radiation is a complete carcinogen, capable of initiating, promoting, and progressing cellular changes that lead to cancer. Therefore it is possible to see radiation-induced neoplasia develop in a radiation treatment field. It appears that orthovoltage radiation and radiation with high linear energy transfer (high-LET) such as neutrons result in carcinogenesis at a higher frequency than the megavoltage photons typically used in veterinary radiation therapy.10 Other factors that influence the risk of radiation carcinogenesis include the age of the patient (young patients are more likely to develop subsequent tumors) and the tissues irradiated. Certain tissues are also more prone to development of radiation-induced tumors, such as the thyroid gland. For a tumor to be considered radiation induced, the following criteria must be met10,11: 1. The malignancy must arise within the irradiated field. 2. Sufficient latency must have elapsed between the time of irradiation and development of the tumor (typically at least 1 year). 3. The original tumor and the new tumor must have different histologic diagnoses. 4. The tissue in which the new tumor forms must have been normal prior to radiation exposure. The overall incidence of radiation-induced tumors in patients treated with radiotherapy is thought to be extremely low (<1% to 2% of patients treated).11 After a tissue or population of cells is exposed to any dose of radiation, a fraction of the cells will be killed. The proportion of remaining cells is known as the surviving fraction (S). The sensitivity of a tumor or tissue to radiation can be shown as a graph of the radiation dose (D) versus the surviving fraction (Figure 12-2).12 The relationship between a dose of radiation and the surviving fraction of cells is commonly described by the linear quadratic equation: where S is the surviving fraction at a dose (D).13 Alpha (α) and beta (β) are constants that vary according to the tissue with α corresponding to the cell death that increases linearly with dose and β corresponding to cell death that increases in proportion to the square of the dose (also known as the quadratic component). The α/β ratio is a useful number that is the dose in Gy when cell kill from the linear and quadratic components of the cell survival curve is equal. Cells with a higher α/β ratio have a more linear appearance when plotted on a log scale, and cells with a low α/β ratio have a parabolic shape. The α/β ratio is also an important description of the radiosensitivity of a cell. At low-dose fractions, tissues or cells with low α/β ratio are relatively radiation resistant compared to tissues or cells with high α/β ratio. It has been suggested that tissues and cells with low α/β ratios have a greater capacity for repair of sublethal radiation damage. Sublethal radiation damage is defined as damage that can become lethal if it interacts with additional damage. Sublethal damage repair is the reason that cell survival increases when a radiation dose is split into two fractions separated by a time interval. Most early responding tissues and tumors have a high α/β ratio, whereas late-responding tissues have a low α/β ratio.13 There are some tumors that may have a low α/β ratio, which can influence the optimal radiation prescription in terms of total dose, time, and fractionation. Tumors that may have lower α/β ratios include melanoma, prostatic tumors, soft tissue sarcomas, transitional cell carcinoma, and osteosarcomas.14–16 Palliative radiation therapy is commonly used in human medicine, and its use in veterinary medicine has increased in recent years. Palliative radiation is generally hypofractionated compared to curative intent protocols, often administered in larger doses per fraction (6 to 10 Gy per fraction) in 1 to 4 total fractions once or twice weekly. Conversely, palliative therapy can be delivered in conventional doses or modestly hypofractionated daily or twice daily for a short but intense treatment regimen. The goal of palliative radiation therapy is not to provide long-term or definitive tumor control; rather, it is intended to relieve pain or improve function or quality of life in patients in which other factors (e.g., advanced metastatic disease) are likely to lead to early demise. Palliative radiation therapy has been used most often for metastatic or primary bone tumors, principally canine osteosarcoma (see Chapter 24). Palliative therapy is more convenient for the owner, and the cost is modest compared with curative radiation therapy because fewer fractions are administered. It is, however, important to remember that palliative radiation is not a substitute for curative-intent protocols despite the convenience. Some palliative therapy protocols may have an increased probability of causing late radiation effects but because they are prescribed to patients that have a poor long-term prognosis, these late effects may not have time to manifest. Curative-intent radiation protocols require strict adherence to radiation biologic principles; palliative radiation protocols, on the other hand, are far more flexible. As in human hospital settings, the protocols may vary dramatically from radiation center to radiation center. Advances in treatment planning and imaging over the past decade have led to the development of image-based, three-dimensional (3D) conformal radiation therapy (3DCRT), which permits better conformity between the irradiated high-dose volume and the geometric shape of the tumor (Figure 12-3). 3DCRT requires importation of CT, MRI, or positron emission tomography (PET) imaging into the treatment planning system. The animal must be positioned for the imaging in a fashion that can be replicated precisely on a day-to-day basis for treatment. Alpha cradles, acrylic face masks, bite plates, and/or Vac-Lok Cushions (Figure 12-4) often are used as positional aids. The radiation oncologist identifies important normal tissue structures, as well as the gross tumor volume (GTV), clinical target volume (CTV), and planning target volume (PTV) on these images. By definition, the GTV only includes gross tumor, and the CTV includes the GTV plus an expansion based on the known clinical behavior of the specific tumor to account for regional microscopic disease. For example, the CTV expansion for a sarcoma is generally larger than for a carcinoma. If the patient has had cytoreductive surgery and only microscopic tumor remains, there is no GTV, and the CTV is based on the scar, regions of surgical disruption, and an expansion for microscopic disease beyond the surgical site. In addition to the GTV and CTV, the PTV includes expansion for an internal margin (IM) that accounts for variations in size and shape relative to anatomic landmarks (filling of bladder, respiratory movements) and set-up margin (SM). The SM accounts for uncertainties in patient positioning and alignment during planning imaging and subsequent treatments. The better the immobilization device, the smaller the SM expansion can be. SM expansions will vary based on the location of the tumor because some sites such as the head are more amenable to rigid immobilization devices such as bite blocks, which provide better replicability. The IM expansion is impacted more by the radiation therapy device. Machines with on-board-imaging devices such as kV x-ray or cone-beam CT (CBCT) can have more confidence in smaller IM expansions. Decreasing the PTV expansion by using good immobilization and available imaging impacts the volume of normal tissue treated and is a key component to successful 3DCRT and is critical to other advanced treatment modalities. More sophisticated beam shaping is performed by taking advantage of fixed multileaf collimators or custom-made blocks. A major advantage of 3DCRT is that dose-volume histograms can be obtained for the tumor and normal tissue structures. This provides a quantitative method of evaluating treatment plans and enhances quality assurance. Defined dosimetric parameters may be useful predictors of outcome. IMRT and related modalities such as tomotherapy allow even greater sculpting of the radiation dose. These modalities require strategies for patient positioning and immobilization. IMRT requires a specific treatment planning system that uses inverse planning. Inverse planning requires that the various tumor structures (GTV, CTV, and PTV), as well as critical normal tissue structures, be identified and contoured into the planning system. Optimization objectives for each structure are entered, and a sophisticated algorithm attempts to meet all objectives. This is the standard of care for treatment of prostate tumors, head and neck cancers, vertebral cancers, some brain cancers, and pelvic cancers in humans. A major benefit associated with IMRT is that the dose to adjacent normal tissue structures can be minimized, dramatically reducing acute effects. Patients are more comfortable and require less pain medication.17 In addition, the tumor dose can be increased without exceeding normal tissue tolerance, presumably leading to improved tumor control. Fractionation schedules similar to those for conventional radiation therapy are used. Tomotherapy, a form of IMRT that uses a helical delivery system to sculpt the beam, is also being used in veterinary medicine.18,19 IMRT is proving useful for the treatment of tumors or tumor beds with complex geometry located near important normal tissues, such as nasal tumors, oral tumors, urogenital tumors, and in cats, vaccine-associated sarcomas.17 SRT describes an emerging field in radiation oncology that uses advanced technology to achieve a different biologic approach. It was originally coined stereotactic radiosurgery (SRS) when the gamma knife was first developed for treatment of inoperable brain tumors in the 1950s by Lars Leksell.20 The gamma knife uses hundreds of small cobalt sources that can converge in 3 dimensions to focus precisely on a small volume of tumor. The gamma knife initially required a rigid frame-based positioning device that was bolted into the patient’s skull prior to imaging and treatment planning. Therefore it was primarily used as a single fraction treatment. CyberKnife is a robotic radiosurgery system incorporating a small linear accelerator on a movable robotic arm, integrating advances in robotic technology and real-time computer-tracking technology.21 The small linear accelerator is moved around the patient and tumor by the robotic device, while the tracking system verifies position by tracking fiducial markers placed in the tumor prior to treatment planning. This frameless tracking system makes repeat fractions feasible, allowing the benefits of modest fractionation. It also makes treating tumors in other parts of the body possible. SRT can also be delivered by specially designed linear accelerators that have designated beams with attributes conducive to small fields and on-board imaging (OBI) capability. Patients are placed in positioning devices such as bite blocks, face masks, and Vac-Lok cushions prior to imaging. For tumors associated with bony structures such as nasal or brain tumors, orthogonal kV images are obtained and the operating software allows the patient’s treatment position to be “matched” to the original imaging positioning. The couch will then make an automatic adjustment. For tumors that may move relative to adjacent bone, CBCT is used and a 3D match is used to verify tumor position.22 The benefits of accelerator-based SRT are that treatment times are generally short, almost any tumor location is accessible, and tumors with larger volumes can be treated. Radiosurgery has been used on a limited basis in veterinary medicine for brain and bone tumors23–25 and more recently for nasal tumors, multilobular osteochondromas, pituitary tumors, thyroid tumors, heart-based tumors, sarcomas, and tumors in the pelvic canal (personal communications, James Custis, Susan LaRue).26 Technologic advances such as those described previously are likely to become available on a limited basis over the next decade. An important consideration is identifying which tumor types will benefit most from such approaches.
Radiation Therapy
Biologic Principles of Radiation Oncology
Repair of Radiation Damage
Time, Dose, and Fractionation
Acute and Late Effects
Radiation-Induced Neoplasia
Cell Survival After Irradiation
Palliative Radiation Therapy
Radiation Therapy Equipment
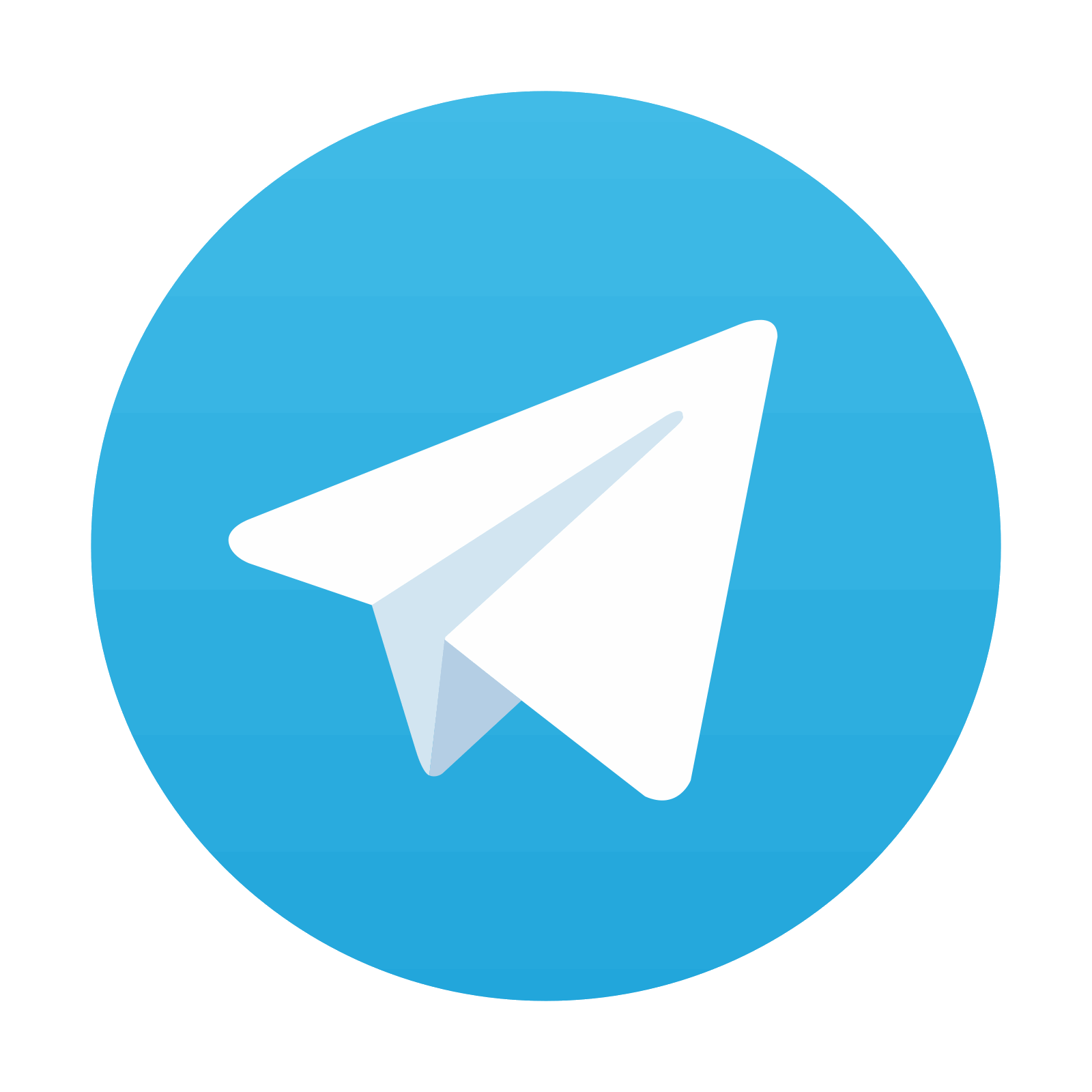
Stay updated, free articles. Join our Telegram channel
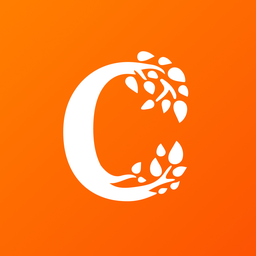
Full access? Get Clinical Tree
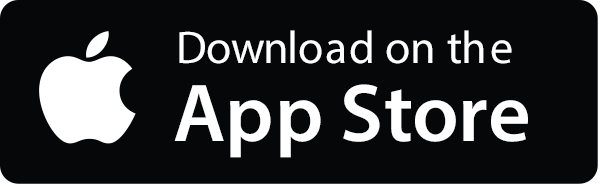
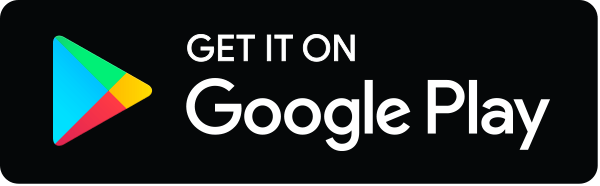