5 Raafat M. Fahmy and Marilyn N. Martinez Pharmaceutics is an interdisciplinary science that involves the integration of physical pharmacy, pharmaceutical formulation, manufacturing technology, dispensing pharmacy, and the laws of pharmaceutics. Irrespective of whether a drug product is intended for use in humans or veterinary species, the same factors influence the process of dosage form development and quality control considerations. Many of these concepts were introduced in Chapters 2 and 3 of this text and will be expanded upon here to give a perspective on how dosage forms influence drug disposition and activity. These factors include: The contents of this chapter provide an overview of each of these points with respect to their relationship to drug products approved for veterinary use. To that end, pharmaceutic principles are discussed from the perspective of the design of drug delivery systems (dosage forms), the formulation and manufacturing variables influencing in vivo and in vitro product performance, and the product understanding necessary to assure that the dosage form is effective, safe, and stable. It should be noted that for those readers interested in learning current recommendations for testing methods for assessing drug intrinsic solubility, the USP has published a general chapter (<1236>) on this topic. The formulation challenges impacting the animal health pharmaceutical industry parallel those encountered during the development of human pharmaceuticals. In addition, the selection of dosage forms and their corresponding formulations reflect the specific needs of the target animal species, potential breed variation that can influence the rate and extent of drug exposure, the variation in body sizes, species feeding habits, logistical challenges associated with product use, cost to the end user (a question of particular concern for products associated with maintaining herd health), environmental safety, animal disease states (and their impact on drug intake or drug pharmacokinetics), and human food safety (for products intended for administration to food producing animals). To accommodate this diverse range of needs, veterinary medications can be manufactured in a variety of forms including: Irrespective of the dosage form, it is important to understand the physical and chemical properties of an API prior to product development. Most APIs in use today are solid materials (pure chemical compounds of either crystalline or amorphous structure). In the case of biomass products, the production of the API and related fermentation products need to be carefully controlled. The purity of the chemical substance is essential for its identification and for determining its chemical, physical, and biological properties. API physicochemical properties may include rheology, particle size, crystalline structure, melting point, and intrinsic solubility, solid-state properties, partition coefficient, pKa, stability, and ionization constant(s) (the extent to which the acid or base ionizes once put into solution). As described in Figure 5.1, integrating our knowledge of the chemical, physical, and biological properties (the latter including the ability of the API to reach its site of action and to generate a biological response) into drug discovery and product development allows for defining the product’s Critical Quality Attributes (CQAs) and for formulation optimization. Figure 5.1 Integration of the physical, chemical, and biological properties of an active pharmaceutical ingredient (API). Many APIs are precipitated during their final stage of synthesis, thereby forming a specific crystalline state. In turn, the crystalline state is a function both of the solvent system used during the initial solubilization process and the conditions of the subsequent drug precipitation. Condition variables of importance include temperature, pressure, rate of cooling, and time. The resulting crystal lattice is comprised of repeating units called unit cells, and all unit cells within a specific crystal are identical (i.e., the same size and arrangement of atoms). Higher levels of energy are required to break strong vs. weak crystal lattices (i.e., characterized by a high melting point). As a result, these more stable crystalline forms typically exhibit low aqueous solubility. Conversely, the amorphous state is characterized by random and irregular positioning of the atoms and a low melting point. An amorphous chemical substance contains no distinct crystalline lattice and therefore is typically the most soluble form of a chemical substance. Although a low melting point is associated with higher aqueous solubility, it also tends to be less stable. In fact, one of the problems associated with in vivo drug precipitation (solubilized drug that recrystalizes in vivo) is that the administered amorphous form (which was soluble) precipitates into a higher energy form, thereby leading to bioavailability (solubility) problems. It is for this reason that much attention is currently being given to the development of drug formulations that support in vivo supersaturation (i.e., the drug remains in solution at concentrations greater than its thermodynamic equilibrium solubility), thus leading to enhanced intestinal drug absorption (Gao and Shi, 2012). Polymorphism is a term used to describe the phenomenon where a drug can exist in more than one crystalline form (i.e., with different lattice arrangements). The difference in molecular arrangement is illustrated in Figure 5.2. The resulting crystal lattice can profoundly influence crystal properties. Polymorphs of an API differ in their solubility, dissolution rate, density, hardness, and crystal shape (Sun, 2009). Figure 5.2 An example of two polymorphic forms of the same compound. An additional consideration is that crystalline forms can exist as anhydrous configurations or as hydrates or solvates. Solvates are crystalline solid adducts containing either stoichiometric (fixed integral ratio) or nonstoichiometric amounts of a solvent incorporated within the crystal structure. If the incorporated solvent is water, then the crystalline form is known as a hydrate. The state of molecular hydration can affect solubility. In general, the anhydrous form of the drug is more soluble than the hydrate due to the stability of the crystalline arrangement (i.e., there is a higher melting point associated with hydrates). For example theophylline is available as either the hydrate or as the anhydrous form. Similar differences in polymorphic forms are also seen with penicillin and caffeine. For each of these compounds, the various polymorphs are characterized by differences in their respective in vitro dissolution characteristics, with the solubility and dissolution rate of the anhydrous crystal exceeding that of the hydrate. Furthermore, the packing geometry leads to differences in their respective lattice energies. Packing geometry is the orientation or conformation of the molecules at the lattice sites, which leads to differences in the amount of energy required to break the crystalline lattice structure. Thus, polymorphic forms usually exhibit different physicochemical properties, including melting point, bulk density, powder flowability, compressibility, hardness, crystal shape, and solubility. Since at least one-third of all organic compounds exhibit polymorphism, polymorphic screens are an important tool in the pharmaceutical scientist’s arsenal. Evaluation of crystal structure, polymorphism, and solvate form is an important preformulation activity. The changes in crystal characteristics can influence bioavailability and chemical and physical stability. Techniques used to determine crystal properties include hot stage microscopy, thermal analysis, infrared spectroscopy, near infrared spectroscopy, and X-ray diffraction. When considering particle geometry, parameters of importance include size and shape. In turn, these influence packing properties and density. Particle size can affect such CQAs as dissolution rate, content uniformity, taste, texture, color, and stability. The extent to which some of these factors are influenced by particle size is determined, at least in part, by the aqueous solubility of the API (where particle size is more important for the dissolution of poorly soluble compounds) and by the product formulation (for content uniformity). Particle size reduction is usually accomplished by shearing, impact, attrition, or compression (dry-milling processes). An excellent review on the importance of particle size specifications was provided by Sun et al. (2010). A reduction of particle size increases the particle’s surface area, decreases the diffusion (or unstirred) boundary layer (the stagnant layer of solvent surrounding the particle), and therefore may enhance drug solubility. A further discussion of this relationship is provided in this chapter’s section on solubility. However, while an increase in surface to volume ratio via a decrease in particle size can enhance API solubility, there are situations whereby decreasing the particle size can have the opposite effect. For example, the cohesivity of particles (tendency to stick together) increases as the particle size decreases. This is a major issue with nanoparticle formulations. Furthermore, particle size governs sedimentation, the formation of aggregates (also known as flocculation, where smaller particles settle faster than do the larger particles) and crystal growth. Crystal growth occurs when smaller particles dissolve and subsequently recrystallize, whereupon they adhere to larger particles, thereby forming larger crystals (a phenomenon referred to as Ostwald ripening). The seeding effect of larger particles on crystal growth is termed nucleation, and a formulation strategy to suppress nucleation is the use of colloid systems (Ravin and Rodebough, 1980). With regard to manufacturing processes, particle size distribution will affect powder flow. Particles ≥250 μm are usually relatively free flowing while very fine particles (below 10 μm) tend to be extremely cohesive and flow poorly. The converse is true with regard to blend uniformity where smaller particles typically facilitate content uniformity. Particles can be characterized by their geometric shape, surface characteristics, and whether they are single particles or are associated as multiparticulate bodies. Shape can influence mixing and tableting processes. The simplest way to describe the shape of a particle is to use a metaphor or to compare it to a commonly known object as in such terms as “egg-shaped” and “granular.” The following are some of the commonly used descriptors of particle shape: The pKa of a drug influences lipophilicity, solubility, protein binding, and membrane permeability. Consequently, it affects such PK characteristics as absorption, distribution, metabolism, and excretion (ADME). Typically, the unionized molecule exhibits greater lipid solubility than does its ionized form. Accordingly, the unionized molecule tends to be the form that can most readily cross biological membranes. Conversely, the ionized form is typically responsible for product dissolution in biological fluids (Kerns and Di, 2004; Avdeef, 2001). Based upon our knowledge of the drug pKa and the surrounding pH, the relative amount of ionized and unionized drug in solution at a particular pH can be determined. The relationship between pKa, pH, and the relative amounts of ionized versus unionized drug can be estimated by applying the Henderson–Hasselbalch (HH) equation introduced in Chapter 2 (Equations 2.2 and 2.3; Figure 2.3). The underpinnings of the HH equation are described by understanding the behavior of weak acids and bases (Figure 5.3). Figure 5.3 The relationship between the preponderance of the ionized versus unionized form of a compound as a function of pKa versus pH for weak acids and weak bases. Strong acids and bases are those that completely dissociate into ions once placed in solution. Weak acids and bases, however, do not fully dissociate in the solution. Weak acids have a higher pH than strong acids because weak acids do not release all of their hydrogens. The acid dissociation constant tells us the extent to which an acid dissociates. The ionization of a weak acid is represented by (Equation 5.1): The dissociation constant, Ka can be calculated as follows (Equation 5.2): The HH equation for a weak acid is described as follows (Equation 3): where A− is the ionized form of the weak acid and HA the unionized form of the drug. Accordingly, when the pH exceeds the pKa, the ionized form of the drug will predominate. Conversely, for a weak base, pH is defined as follows (Equation 5.4): where B is the unionized form of the weak base and BH+ is the ionized form of the drug. Accordingly, when the pH exceeds the pKa, the unionized form of the drug will predominate. Based on Equations 5.3 and 5.4, we can calculate the percentage of drug existing in the unionized form at a given pH (Equation 5.5): where charge = −1 for acids and 1 for bases. In most situations, drugs must cross biological membranes before entering the bloodstream. Since biological membranes are lipophilic in nature, there is the competing challenge of solubilization in an aqueous environment versus the need to partition across the lipophilic biological barrier. This fine balance necessitates that the drug can readily pass between the aqueous and lipid phases to insure drug absorption. Figure 2.2 illustrates this concept. This capability is described by the partition coefficient, P, which characterizes the relative oil versus water affinity of a compound when it exists in its unionized form. The determination of P (or Log P) involves the addition of the API to a separatory funnel that contains a mixture of two immiscible solvents. Drug molecules then distribute between these two layers until equilibrium is established. The resulting estimate, the Log P value, is often used to predict API absorptive capability. If the compound is acidic or basic, the pH is adjusted to insure the compound is neutralized. Once equilibrium has been achieved, the compound is quantified in each solvent, typically via ultraviolet/visible spectroscopy or by titration, and the logarithm of the concentration ratio is calculated. The measurement is expressed with the following Equation 5.6: The resulting Log P value specifies whether the measured compound is hydrophobic or hydrophilic. The reason why octanol is selected as the lipophilic solvent is because it acts both as a hydrogen bond acceptor and donor, thereby simulating the properties of biological membranes (Fletcher et al., 2013). The interpretation of Log P values relative to permeability predictions are as follow (Lipinski et al., 2001): Another common measure for lipophilicity is the distribution coefficient, Log D. Log D accounts for the existence of molecules both in its ionized and unionized forms. Accordingly, measurements of Log D are generated across a range of pH values. For unionizable compounds, Log P = Log D at any pH. Amongst the different pH values, typically the most interesting is pH 7.4, the physiological pH value. Each pure substance is characterized by a specific melting point or melting range. The presence of impurities results in a change in the melting point. This phenomenon is commonly used to determine the purity of a drug substance. In addition to its importance regarding the determination of impurities, melting point of the pure API needs to be considered during the manufacturing process. In particular, it can influence the appropriateness of using hot melt extrusion techniques (e.g., chewable dosage forms) or the stability of the product when encountering the heat produced during process granulation. Rheology is a study of the flow and deformation characteristics of a material (liquid or semisolid) when subjected to an applied force. Deformation is the transient change in shape of matter. If the shape of the material reverts to its original state after the stress is removed, the material is said to be elastic (e.g., a rubber ball). Conversely, if the matter does not deform, it is considered to be rigid. Material flow is dependent on the ability of the material to deform. For example, if toothpaste is placed into a square container and that container is subsequently squeezed, displacing toothpaste into a round container, the toothpaste would take on the shape of the new container. This movement of the toothpaste is defined as flow. Rheologic measurements are used to characterize the ease of flow and the ability of the material to deform. These are important characteristics in the manufacturing process as it influences the ability of the product to maintain its shape after extrusion or the problems that will be encountered during product mixing and storage. The rheological properties of an API can also influence the homogeneity of a formulation. The ability to deform determines the magnitude of internal friction generated in response to an external stress. In this regard, the greater the deformation properties of the drug, the smaller the friction and the higher the flow rate in response to any given external stress. The measure of resistance to flow is defined as the viscosity of the fluid. A fluid is considered to exhibit Newtonian flow if the viscosity does not change in response to the application of sheer. An example of a Newtonian fluid is water. In contrast, some non-Newtonian materials (such as yogurt, latex or acrylic paints) exhibit thixotropy (a reduction in viscosity in response to an increase in relative flow velocity by processes such as stirring) while others (e.g., thickening agents) exhibit an increase in viscosity in response to an increase in sheer stress (Wood, 1986). Stability of the drug substance should be determined in the early phases of product development. A drug product is considered unstable when the API loses sufficient potency that it will adversely affect the safety (e.g., potential for toxic degradants) or efficacy within a timeframe deemed unacceptable for the desired shelf life. Stability testing provides information on the API behavior under a variety of environmental factors such as temperature, humidity, and light. It also establishes a retest period for the drug substance, shelf life for the drug product, and leads to recommendations with respect to drug and drug product storage conditions. Oftentimes, degradation products can be predicted a priori from the chemical structure of the API. For example, esters and amides are sensitive to hydrolytic degradation while acridans and catecholamines are sensitive to oxidative degradation. Degradation mechanisms include: Stereochemistry is a study of the structural arrangement of the atoms within the molecule or the functional groups in the molecule. Stereoisomers are characterized as having the same composition but differences with respect to its spatial orientation. There are two kinds of stereoisomers: enantiomers and diastereomers. Enantiomers are non-superimposable mirror images. In contrast, diastereomerism occurs when two or more stereoisomers of a compound have different configurations at their stereocenters and are not mirror images of each other. Diastereomers generally have different physicochemical characteristics and therefore are considered to be different compounds. Whether to formulate a product using a racemate or an enantiomer of a chiral drug (i.e., a drug containing a tetrahedron that results in the presence of molecular forms that are nonsuperimposable mirror images) depends on such considerations as the relative pharmacodynamic activity and the potential toxicity (or side effects) of the individual enantiomers, their pharmacokinetic profiles, and, importantly, the proportions of the individual enantiomers that may form over time in the target animal species (i.e., in vivo chiral inversion) (Baggot, 2007). Furthermore, there can be occasions when one enantiomer of an API is active but the other is not. Use of the racemic mixture leads to half of the administered API being without clinical effect. An example of this is omeprazole versus esomeprazole (Andersson, 2004). The stereochemistry of the API can also lead to differences in the product dissolution rates. For example, cellulose derivatives, ascorbic acid, mannitol, sorbitol, lactic acid, and malic acid, are examples of chiral excipients that can interact with drug enantiomers and produce stereospecific release and absorption from the formulation. Enantiomers are considered to be the same compound but are designated with regard to its geometric configuration. Generally, this is the designation of R or S, reflecting the orientation of each chiral center. R and S forms of a compound can have different biological activities. For example, biological differences due to enantioselectivity are provided in Table 5.1. Table 5.1 Biological differences between S and R forms of various active pharmaceutical ingredients An additional geometrical term used to describe the entire molecule is dextrorotatory (D) or levorotatory (L), depending on its exact substituents. D and L symbols are associated with absolute configuration of a molecule, and this designation is based on the absolute configuration of the dextrotatory and levorotatory forms of glyceraldehyde. The D/L system remains in common use in certain areas of biochemistry, such as amino acid and carbohydrate chemistry. Most naturally occurring carbohydrates are nearly all D while most amino acids are L. An enantiomer can be named by the direction in which it rotates the plane of polarized light. If it rotates the light clockwise (as seen by a viewer towards whom the light is traveling), that enantiomer is labeled (+). Its mirror image is labeled (−). The (+) and (−) isomers have also been termed d- and l-, respectively (for dextrorotatory and levorotatory). Naming with d- and l- is easy to confuse with D- and L- labeling and is therefore strongly discouraged by IUPAC (Klink et al., 2001). Solubility can be defined as the ability of a substance (the solute) to dissolve in a fixed amount of solvent (e.g., water or buffered solution) to form a saturated solution under a specific temperature and pressure. When the solvent is saturated with solute, the concentration of solute is called the saturation concentration. In the literature, the term saturation concentration (CS) is often used interchangeably with the term solubility. Intrinsic solubility for an ionizable molecule is defined as the concentration of the unionized molecule in a saturated aqueous solution, existing in a thermodynamic equilibrium at a given temperature (Horter and Dressman, 2001). In contrast, when an excess amount of solid is inserted into the solvent, under defined temperature and after sufficient stirring time, the amount of dissolved solute may be initially high but then will reduced to its thermodynamically stable value over time. The time it takes for such a reduction in solubilized concentration depends on several factors, and varies from seconds to months. When the obtained saturation concentrations are much greater than the thermodynamically stable values, the term apparent solubility is applied. The term apparent solubility refers to the metastable or dynamic solubility of the materials rather than to a thermodynamically stable state (Mosharraf and Nyström, 2003). The relationships between dose, dissolution characteristics, and drug solubility can be described in Equation 5.7 as follows (Lobenberg and Amidon, 2000). where The corresponding ratio of dose to dissolved drug i.e., the dose number, D0, is described by: where M is the dose of the drug and V0 is the volume of fluid consumed with the dose. Within the pharmaceutical sciences, the focus is on aqueous solubility because water is the biologically relevant solvent. However, it should be kept in mind that, as discussed later in this section, the API must cross biological membranes to exert an effect (e.g., enterocyte, endothelial cell, targeted host cell or a pathogen). Therefore, the relative solubility (partitioning capability) between a hydrophilic and hydrophobic solvent (e.g., octanol versus water) is also a critical consideration when developing drug candidates. Aqueous solubility is controlled by two opposing interactions. Favoring API solubilization is the interaction between a molecule of the API and the solvent. Counteracting aqueous solubilization is the affinity of the solute for itself. It is the greater of these two effects that dictates the maximum amount of drug that will dissolve per unit volume of solvent. Because many of the more recent drugs exhibit very strong self-affinity (low aqueous solubility), much work is currently being conducted on mechanisms to reduce the strength of these internal interactions, thereby enhancing solubility in aqueous media (Delany, 2005). Pharmaceutical techniques explored for achieving this enhanced aqueous solubility include formulation adjustments or molecular modifications (e.g., new salt forms). Assessment of drug solubility is not necessarily straightforward as there are numerous variables that affect solubility results. We have already discussed some of these factors, including drug pKa, polymorphisms, particle size and shape, chemical purity, and the potential for molecular stability problems when present in its dissolved state. Several software programs are capable of synthesizing information on these variables into solubility and permeability predictions. Such information can be invaluable both for the formulation chemist and for the pharmacologist who seeks to understand how target animal species-specific in vivo conditions can alter drug product dissolution and drug membrane permeability. For any set of API chemical characteristics, the solubility of the API in aqueous solutions can be influenced by: Surfactant: Surfactants are typically organic compounds that contain both a hydrophobic head and a hydrophilic tail. As a result of their structure, they lower the surface tension (or interfacial tension) between two liquids or between a liquid and a solid. In so doing, the surfactant can act as detergents, wetting agents, emulsifiers, foaming agents, or dispersants. Examples of surfactants include polysorbates, polyoxyethylated castor oil, polyoxyethylated glycerides, lauroyl macroglycerides, and mono- and difatty acid esters of low molecular weight polyethylene glycols (e.g., lipids, surfactants) which are miscible in aqueous fluids, serving to enhance the drug–water interaction. As discussed by Martinez and Amidon (2002) as well as in Chapter 2 of this text, molecular movement across lipid bilayers, such as those within biological membranes, is extremely complex due to the regional differences in membrane polarity, hydrophobicity, and density. Generally, the bilayer can be divided into four distinct regions. These are: Owing to the anatomy of this complex barrier, drug permeability is not a simply two-step process of solubilization and diffusion. Rather, it represents a spectrum of complex molecular events. Accordingly, membrane penetration reflects the interaction of several factors, including molecular size (negatively correlated), lipophilicity (positively correlated), polar van der Walls surface area (negatively correlated), and the molecular flexibility (intramolecular hydrogen bond formation). Molecular flexibility and the corresponding ability to undergo conformation changes can significantly affect the polar surface area of a molecule. The polar surface area and nonpolar surface area are predictors of intestinal permeability, being respectively inversely and directly related to membrane permeability. Another important variable is the strength of the hydrogen bonds formed between the molecules of water and solute. It is generally assumed that these bonds must be broken (desolvation) before the solute can traverse the biological membrane. Impurity can affect stability, appearance, and API toxicity, (e.g., aromatic amines, suspected of being carcinogenic). In most cases, the impurities (reactive species) consist of water, small electrophiles (such as aldehydes, carboxylic acid derivatives, and peroxides), and metals. Water can hydrolyze some drugs. Aldehydes and carboxylic acids can form molecular adducts. Peroxides can oxidize some drug. Metals can catalyze oxidation, hydrolysis, and other degradation pathways (Fahmy et al., 2008). Impurities can lead to altered bioavailability, toxicity, or a reduction in effectiveness. There are more than 800 excipients currently used in marketed pharmaceutical products within the United States (human and veterinary). This number is expected to increase to accommodate the evolution in therapeutic entities such as those associated with gene and cell therapy or the development of new drug delivery platforms (Bhattaryya et al., 2006). The purpose of excipients varies as a function of the drug, dosage form, and the intended in vivo release characteristics. Pharmaceutical excipients may be used for any one (or combination) of the following objectives: Both synthetic and natural ingredients have been used as excipients in pharmaceutical products. Examples include: For excipients not described in any pharmacopoeia, specifications should include physical characterization, identification tests, purity test, assay, and impurity tests. As with human pharmaceuticals, all excipients used in the veterinary medications are manufactured and supplied in compliance with compendial standards and should comply with the United States Pharmacopeia (USP) or National Formulary (NF) monographs or with any other recognized pharmacopeia. The Food and Drug Administration (FDA) approves all excipients that are included in pharmaceutical products. A certification of analysis (COA) must confirm that excipients are of nonanimal origin. If this is not the case, the regulatory agency requires documentation to demonstrate freedom from viral and transmissible spongiform encephalopathies (TSE) and bovine serum encephalopathy (BSE) risks. Excipients from an animal origin should be identified. The genus, species, country of origin, source (e.g., pancreas), and manufacturer or supplier should be clearly indicated. Furthermore, for excipients derived from ruminant materials, the application should state whether the materials are from BSE countries as defined by the US Department of Agriculture (9 CFR 94.11). Guidance is available from the FDA on The Sourcing and Processing of Gelatin to Reduce the Potential Risk Posed by Bovine Spongiform Encephalopathy (BSE) in FDA-Regulated Products for Human Use. The potential adventitious agents should be identified, and general information regarding control of these adventitious agents (e.g., specifications, description of the testing performed, and viral safety data) should be provided in this section. Details of the control strategy and the rationale for the controls should be provided. Additional guidance is available in International Conference on Harmonization (ICH) guidelines: It is critical that the API and excipients do not interact with each other and/or the container over time. If interaction occurs, product quality and performance can be compromised. An additional consideration is the safety of the excipient. The safety of a particular component of human food and drugs does not necessarily insure its safety for consumption by a veterinary species. For example, xylitol, a popular human sugar substitute was found to be highly toxic, or even fatal, when given to dogs. Some excipients have their own inherent activity that can influence drug pharmacokinetics. For example, the vitamin E derivative, α-tocopherol polyethylene glycol 1000 succinate (TPGS), when administered at low concentrations, appear to enhance the secretion of chylomicrons by enterocytes. In turn, this can lead to a significant enhancement of intestinal lymphatic transport of lipophilic drugs. In addition, several reviews show that a number of excipients can influence the in vivo and in vitro activities of a variety of cytochrome P450s (CYPs) or membrane transporter activities (Ren et al., 2008; Buggins et al., 2007). For any given dosage form, the excipient is selected to achieve a specific function. While there can be multiple choices of excipients, the final selection is based upon its specific properties and how it will impact product in vivo performance, stability, and ease (and uniformity) within the manufacturing process. In the next section, we provide a brief overview of the functional considerations that go into the determination of excipients included in solid dosage forms. The following are some examples of the common excipients and their functionalities used in manufacturing different veterinary dosage forms. Examples of commonly used dosage form-specific excipients are described below. Diluents are added to a formulation to increase the bulk volume of the API, making the size of the tablet suitable for handling. They also can improve the product manufacturing properties (e.g., powder flow, compaction properties, and homogeneity) and product quality, such as content uniformity, in vivo disintegration and dissolution, tablet integrity, friability (the ease with which tablets can break into smaller pieces, promoting more rapid dissolution), or physical and chemical stability. The selection of diluents depends on the type of processing and the plasticity (the capability to be deformed) of the materials to be used. Examples of diluents used in veterinary medicine include: microcrystalline cellulose (MCC), lactose, dicalcium phosphate (Di-Cal), mannitol, sucrose, and partial pregelatinized starch. Binders are used for the purpose of holding together the ingredients in a formulation, thereby ensuring that granules and tablets can be formed with the required mechanical strength. It also affects the disintegration rate, dissolution rate, hardness, and friability (Jayesh and Manish, 2009). Examples of excipients that are added to a formulation in a solid form include hydroxypropyl methylcellulose, polyvinylpyrrolidone (PVP), starch, and polyethylene glycol. Alternatively, it could be dissolved in solvents and added to the powder to form wet granulation such as gelatin, PVP, starch, sucrose, and polyethylene glycol (PEG). Disintegrants are added to promote moisture penetration into the dosage form matrix, thereby ensuring that it will break into small fragments. The formation of smaller tablet particles allows for an API to undergo a more rapid dissolution. Disintegrants can be added to tablets and hard shell capsule formulations (i.e., the drug plus formulation administered as a solid substance enclosed in a capsule). The quality and quantity of the disintegrant affects the product’s dissolution and therefore the bioavailability of the API. Examples of common disintegrants used in veterinary medicine are: starch, (MCC), croscarmellose sodium, and crosspovidone. Glidants are used to promote powder flow within the manufacturing machinery by reducing interparticle friction and cohesion. Glidant particles coat the surfaces of the larger bulk powder particles (‘host particles’) where they may reduce of surface roughness and attractive forces, or act as moisture scavengers. Examples of glidants are: talc powder, sodium lauryl sulfate, colloidal silicon dioxide, and starch. Lubricants are added to formulations to prevent its sticking to the manufacturing equipment. Although there are both hydrophilic and hydrophobic lubricants, it is the latter that is more effective in achieving the desired manufacturing benefit and therefore is the one generally used in tablet formulations. Commonly used hydrophobic lubricants include magnesium stearate, calcium stearate, and stearic acid. Of particular note for these hydrophobic (waxy) lubricants is that the dissolution rate of poorly soluble APIs is influenced not only by the amount of lubricant added to the formulation but also by the order of its addition relative to the other constituents and the blending time of the lubricant with the other ingredients. This effect appears to be attributable to the smearing of the hydrophobic lubricant (e.g., magnesium stearate) over particle surfaces, resulting in a reduction in product in vivo absorption (Kalyana et al., 2011). Tablet coating is used to improve product appearance, to provide taste/odor masking, to facilitate swallowing, to protect from moisture/light, to improve mechanical strength, and as a mechanism for modifying drug release (e.g., enteric coated tablets). Examples of coating agents include sugar, hydroxypropyl methylcellulose (HPMC), cellulose acetate phthalate (Aquacoat® CPD; enteric), ethylcellulose (sustained release), hydroxypropyl methylcellulose acetate succinate (enteric), and polyvinyl acetate phthalate (enteric). Excipients used in modified release (MR) tablets fall into two broad groups based on whether they are cellulose or acrylic polymers. The most common technique for producing a MR tablet is either through the formation of matrices or by the inclusion of special tablet coatings. The most common excipients for the matrix tablets are sodium carboxymethylcellulose, HPMC high viscosity, a carboxyvinyl hydrophilic polymer, and PVP. The most common excipients for coated tablets are ethyl cellulose (the most frequently used cellulose-based polymer used for extended release) and PVP. These excipients are generally used to increase the mass of a lyophilized product, thereby providing structure to the formulation. Bulking agents are used for low-dose, high-potency drugs (when the API constitutes less than 2% in the formulation) and therefore does not have the bulk necessary to support its own structure. Examples of bulking agents include mannitol, lactose, and sucrose. The choice of buffer depends on the pH stability profile of the API. Buffer selection, along with its concentration is important to avoid API degradation during processing or during storage and reconstitution. Buffer selection also influences the optimization of product solubility. Commonly used buffers in the parenteral formulations are acetate, citrate, tartrate, phosphate, triethanolamine (TRIS), benzoate sodium /acid, boric acid/sodium, arginine, hydrochloric acid, sodium hydroxide, succinate sodium, sulfuric acid, and sodium carbonate. Injectable formulations should preferably be isotonic to prevent osmotic shock at the injection. Examples of commonly used tonicity adjusting agents are alkali metal or earth metal halides such as CaCl2, KBr, KCl, LiCl, NaI, NaBr, NaCl, Na2SO4, or boric acid. Nonionic tonicity agents include glycerol, sorbitol, mannitol, propylene glycol, or dextrose. The typical preservatives used in liquid formulations include a range of antioxidants, antimicrobial agents and chelating agents (Chaubal et al., 2006). These are used to stop the growth of any microorganisms in the drug product. Examples include benzyl alcohol, parabens (methyl, propyl, butyl), benzoic acid, phenol, thimerosal, meta-cresol, benzalkonium chloride, chlorobutanol, glutathione, and others. Chelating agents (substance that bind with free metals such as Cu+, Cu2+, Fe2+, and Fe3+), are generally permitted to be present in trace amounts in the formulation. Since the newly formed complex ions are nonreactive, chelating agents remove the capacity of the metal catalysts to participate in oxidative reactions. Chelating agents also have the ability to enhance antimicrobial effectiveness by forming a metal-ion–deficient environment that otherwise could feed microbial growth. Examples of chelating agents include sodium calcium, ethylenediaminetetra acetic acid EDTA, diethylenetriaminepenta acetic acid (DTPA), calteridol, and disodium EDTA. These are used to prevent or minimize oxidation reactions over the shelf life of the product. The most commonly used antioxidants in sterile formulations are ascorbic acid, acetylcysteine, sulfurous acid salts (bisulfite, metabisulfite), and monothioglyercol. Water is a common solvent system. However, nonaqueous water-miscible agents are used as cosolvents with water to enhance the solubility, stability, and to control drug release from injectable products. Those solubilizing agents, added to the injectable products to enhance the drug solubility into the formulation, are generally classified as surfactants or cosolvents. In contrast to surfactants, which increase API dissolution by reducing the surface tension of the chemical substances, cosolvents are defined as a second solvent that is added in small quantities in conjunction with the primary solvent to increase the API solubility. For compounds with large hydrophobic groups and high Log P values (e.g., 3–4), the use of cosolvents in combination with surfactants may be required (Pramanick et al., 2013). Examples of surfactants are polyoxyethylene sorbitan monooleate (Tween 80), sorbitan monooleate polyoxyethylene sorbitan monolaurate (Tween 20), lecithin, and polyoxyethylene–polyoxypropylene copolymers (Pluronics). Examples of cosolvents include propylene glycol, glycerin, ethanol, polyethylene glycol (300 and 400), sorbitol, dimethylacetamide, and cremophor EL. Complexation is sometimes used to improve the solubility of drug in the solvent, especially water. Cyclodextrins have emerged as very effective additives for solubilizing hydrophobic drugs. In the parenteral dosage forms, modified cyclodextrins such as hydroxypropyl-β-cyclodextrin and sulfobutylether-β–cyclodextrin have been reported to be highly efficient solubilizer/stabilizers (Loftsson et al., 1996). Flocculating agents are used in parenteral suspension injectable products to enhance particle “dispersability,” reduce sedimentation rate in the flocculated suspension, prevent cake formation, and control viscosity. Examples of flocculating agents are potassium/sodium salts such as the chloride, citrate, and acetate (all used primarily in parenteral formulations). Other suspending agents (which may be used in nonparenteral or parenteral formulations) include sodium carboxymethyl cellulose, acacia, gelatin (generally for oral formulations), methyl cellulose, and PVP (Anderson et al., 2006). It should be noted that carboxymethyl cellulose may cause allergic reaction in humans, horses, and cattle (Deweck and Schniede, 1972). These excipients are used to create a homogenous dispersion of solid particles in liquid media. The majority of drugs in aqueous suspensions are hydrophobic and are therefore difficult to suspend. Consequently, they tend to float on the surface of any polar liquid due to entrapped air and poor wetting. The inability of particle wetting reflects a high interfacial tension between material and liquid. Consequently, the interfacial tension must be reduced so that air can be displaced by liquid. Wetting agents are surfactants that lower the interfacial tension and contact angle for solid particles and liquid vehicle. Examples of wetting agents include anionic substances (such as sodium lauryl sulfate, sulfonate sodium, and docusate sodium), cationic substances (such as cetylpyridinium chloride), zwitterions (such as lecithin), and nonionic substances (such as poloxamer and polysorbate). It is the nonionic surfactants that are most commonly used as wetting agents in pharmaceutical suspensions. Commonly used naturally occurring thickening agents are acacia, agar, cellulose, and gelatin. Synthetic thickening agents include methylcellulose, microcrystalline cellulose, and colloidal silicon dioxide. Coloring agents can be classified as soluble in water (dyes) or insoluble in water (pigments). The colors approved for the clear liquid formulations are limited to dyes. Examples include sunset yellow, methyl blue, quinine yellow, and FD&C red. These substances prevent drying or loss of moisture from a product commonly used in semisolid preparations. Examples include glycerin, propylene glycol, and sorbitol. These substances are used to conceal or mask the bitter, salty, or offensive taste or odor of a drug substance. Pharmaceutical dosage forms contain both pharmacologically active compounds and excipients. For any given product, its quality and performance will be a function of the physicochemical properties of the API, the excipients, and the manufacturing process. APIs are formed into dosage-form products before they are dispensed or administered to animals. The API is mixed with excipients, such as binders, fillers, flavoring and bulking agents, preservatives, and antioxidants. These ingredients may be dried, milled, blended, compressed, and granulated to achieve the desired properties before they are manufactured as a final formulation. Examples of the dosage form classifications commonly found within the framework of veterinary medicine are provided below (Figure 5.4). Each dosage form is tailored to meet the needs of the specific animal species, the disease condition (e.g., intramammary) or therapeutic goal (intervaginal implants for estrus control), conditions of use (e.g., in hospital, at home use, mass herd treatment). Figure 5.4 Examples of common veterinary dosage forms as a function of route of administration. Solutions are a clear, homogeneous liquid dosage form that contains one or more APIs dissolved within a vehicle. Solutions are a stable system (i.e., the molecules or the ions do not settle out). Particles are not visible, even under highest magnification, but the solute itself could differentially absorb visible light, leading to color. Depending upon its intended route of administration, the solution may or may not need to be manufactured as a sterile product. In cases when the API is poorly soluble, the formation as a solution may necessitate the addition of solvents such as alcohol, propylene glycol, or glycerin. Formulations for oral solutions include solvents, buffers, preservatives, antioxidants, and flavors. Challenges with the manufacture of a solution include API solubility, formulation stability (due to oxidation, pH, temperature, and light), sterility (for some routes), and the potential for microbial contamination. Quality attributes include appearance, pH, assay, impurities, and microbial limit. A liquid suspension is a dosage form containing solid particles dispersed in a liquid vehicle. Unlike solutions, suspensions are considered to be thermodynamically unstable (i.e., capable of separation). Suspensions are for the administration of insoluble or poor soluble drugs. The suspension system consists of two phases: the internal phase, which contains the solid particles that are characterized by a specific size distribution; and the external phase (suspending medium), which while often aqueous, can also be organic or oily. This is illustrated in Figure 5.5. Figure 5.5 Considerations that need to be applied when formulating a drug product as a suspension. Suspensions can be classified according to their particle size: There are several challenges encountered during the development of suspensions. These include: Formulations for suspension include surfactants, buffers, suspending agent, thickening agents, preservatives, antioxidants, and flavors. When dealing with formulations containing two phases (e.g., solid and liquid), there exists an electrical interactive force. Particles contain an electrical charge in polar solvents. The resulting interactions, the electrokinetic potential measured as the zeta potential, will dictate both in vivo and in vitro particle behavior. Quality attributes thus include particle size distribution, pH, rate of settlement, viscosity, dissolution, zeta potential, and flocculation (formation of aggregates). Within the framework of a suspension, there are several related dosage forms that differ from the perspective of its physical properties, impact on bioavailability, ease of use, and suitability for route of administration (e.g., oral, topical, parenteral). These include: A semisolid dosage form containing a large proportion (20–50%) of solids finely dispersed in a fatty vehicle. Vehicles used in the formulation of paste and gels include aqueous solvents (least expensive and poses minimal toxicity), oil, or organic solvents. Challenges associated with the manufacture of pastes and gels include producing the desired viscosity, cohesiveness, lack of plasticity, and the need to avoid a separation of liquid and solid components of the formulation. Typically, glycerin, glycols, natural or synthetic gums, and polymers are used to increase the product viscosity while balancing formulation plasticity and cohesiveness. To overcome the separation of water from the gel, absorbing materials such as MCC, kaolin, colloidal silicon dioxide, and starch can be used. Preservatives may be added to inhibit microbial growth. A semisolid system in which a liquid phase is constrained within a three-dimensional cross-linked matrix. Suspensions (emulsified liquid dosage form) intended for external application (e.g. calamine lotion, hydrocortisone lotion). A cream is a semisolid emulsion systems (o/w, w/o) containing more than 10% of water. Oil in water creams are more common than water in oil because the former tends to be more comfortable to the user, due at least in part to the property of feeling less greasy. An ointment is a highly viscous or semisolid preparation intended for external application. Tablets are compressed powders prepared by one of three general methods: wet granulation, dry granulation (roller compaction or slugging), and direct compression. Issues associated with manufacturing processes are described elsewhere is this chapter. Tablets can be classified on the basis of route of administration (e.g., oral, sublingual, rectal, vaginal, and subcutaneous implant) or formulation characteristics (e.g., immediate release, MR, effervescent, melt in mouth, chewable, flavored, or fast dissolving). In all cases, the general manufacturing processes, technique used for preparation of tablets, and materials are similar. IR tablets typically dissolve in gastrointestinal contents with no purposefully generated delay in drug dissolution or absorption. MR tablets are manufactured with the purpose of altering the in vivo drug release/dissolution. MR tablets can be in the form of extended or delayed release. Expressions such as “extended release”, “prolonged-action,” “repeat-action,” and “sus-tained-release” have been used to describe these dosage forms. However, the term “extended-release” is used for pharmacopeial purposes. ER tablets are formulated to cause the formulation rather than the API to serve as the rate-controlling factor in drug dissolution/release. In so doing, the API can be released over a prolonged duration of time. For orally administered products other than those intended for gastric retention, the success of the formulation is determined by its ability to be absorbed throughout the GI tract. Thus membrane permeability and GI transit time of the target animal species may limit the utility of these formulations in veterinary medicine. ER formulations can be designed for first-order or zero-order drug release. First-order release is characterized by a specified percentage of the label dose being released over time. A zero-order release of drug is characterized by a specified amount of drug released over time. Chapter 2 should be consulted for more details on the impact of rate-limiting processes on drug pharmacokinetics. Preference is dictated by the absorption properties of the drug and the API exposure–response relationship. The altered drug release characteristics may be achieved through the use of matrix systems (e.g., hydrogels, polymeric matrix, and wax matrix), multiparticulate systems (e.g., granulates, pellets, and beads), polymeric film coating, ion exchange resins, pH-dependent release methods (e.g., enteric coating), and laser drilling. Some of these methods are best achieved by using a capsule rather than a tablet dosage form. Generally, delayed release tablets are designed to constrain the release of the drug substance until the tablet has passed through the stomach. Delayed release tablets are desired when the drug may be destroyed or inactivated by the gastric juice or when it may irritate the gastric mucosa. Delayed release can be accomplished through the use of enteric coatings. The bolus is a very large tablet that is intended to be retained in the rumen. A slow dissolution process and ruminal retention provides an opportunity to achieve prolonged systemic drug exposure. The release of the active ingredient generally relies on erosion, diffusion from a reservoir, dissolution of a dispersed matrix, or an osmotic “driver.” Regurgitation during rumination is prevented by formulating the bolus with a density of ∼3 g/cm3. Critical quality attributes include assay, content uniformity, dissolution, hardness, friability, impurities, and loss on drying. An implant is a small tablet that is manufactured in the same way as a tablet. It is a nonoral dosage form that can remain in the body of the animal for many months. They can be either immediate or MR. It is important to recognize that there are numerous variables that can influence the drug release properties of an implant. For example, drug can diffuse both vertically and horizontally. Therefore, the dimensions of the implant and the diffusivity of the drug within the implant matrix can have significant impact on the in vivo rate and extent of drug release. Furthermore, in some cases, implants are manufactured as multilayer products such that the diffusion of drug through each layer can be controlled. Lastly, there are also implants that work as hydrostatic pumps such that the drug is pushed out as water from the tissues move in (Kleiner et al., 2014). Critical quality attributes include assay, content uniformity, dissolution, impurities, and loss on drying. The development of chewable tablets for dogs and cats started in the 1960s, a time when the companion animal pharmaceutical products were produced with the same or similar design and formulation characteristics as compared to the human pharmaceutical. In the mid-1970, the first “chewable” veterinary tablets were developed for dogs, manufactured using standard pharmaceutical processing equipment. Most of these chewable tablets were manufactured by wet granulation technology using water and corn syrup. The early canine chewable tablet formulations were associated with palatability scores of 70–85%. Feline free choice palatability did not fare as well, often with palatability scores of less than 50%. In the 1980s, milk and cheese flavors were tried in for both dogs and cats. Canine palatability never exceeded 80% free choice acceptance and feline palatability never exceeded 70%. Fruit flavors were common in companion animal chewable oral liquids, but fruits are not part of a companion animal’s natural diet. Garlic, which has long been considered palatable to dogs, resulted in a free choice acceptance of only about 30–60%. When the garlic flavor was removed and a different flavor system used, a free choice level of 95% was achieved. The initial palatability enhancing agents often included animal by-products of questionable quality and reproducibility. Common palatability enhancing agents included bovine pancreas digest, bovine liver extracts, bovine meat by-products, fish meal, fish digest, and other ingredients that were not fit for human consumption. The high fat content of these flavors made them prone to rancidity. Even if stabilized with antioxidants, rancidity was an important stability issue (Fahmy et al., 2008). Furthermore, the animal and fish by-products often had very high microbiological counts (greater than 50,000 cfu/g) and were contaminated with Escherichia coli, salmonella, and other coliform bacteria. This bacterial growth caused the chewable tablets to turn from brown to green and to emit offensive odors (Meijboon and Stronk, 1972). In the early 1990s, the industry started to develop alternative chewable tablet formulations with improved flavoring agents that provided an attractive aroma and taste which led to an increase in free choice acceptance. Today, the new flavoring agents meet human food-grade and/or pharmaceutical grade quality standards, are stable and consistent, and contain negligible to nondetectable levels of bacteria, mold, yeast, and fungi. Chewable tablets can be made by direct compression, wet granulation (using an appropriate solvent), or dry granulation (slugging or roller compaction), extrusion, or produced in a forming machine. “Direct compression” is easy to apply and necessitates minimal capital investment. As this new palatable chewable tablet is formulated, tablet weight and hardness become important variables. For example, when formulating feline chewable tablets, the generalized “ideal” hardness is in the range of 3–4 Kp. All other factors being held constant, as hardness exceeds 6 Kp, the palatability tends to decrease. The identical formulation at 6 Kp tablet hardness may have a 95% free choice acceptance in cats, but only 50% free choice when formulated with a 12 Kp tablet hardness. The excipients are selected so that the blend can be formed into shapes through a forming machine. In this process, the dry components, which include the API, flavor, fillers, binder, lubricant, and any other ingredients, may be milled and blended. The solvents are added and are mixed until a dough is formed that has the desired texture. The dough batch is divided into portions in accordance with the sublot designation of the configuration being manufactured. The dough sublot is transferred to the forming machine hopper, producing a range of shapes or chews and dosage form weights that can be as high as 15 g. Once the chews are formed, they are taken to either an oven at 40–50oC and/or left at room temperature with relative humidity 40–70% (for up to 7 days depend on the nature of the polymer or the formulation) for curing. An example of a chewable forming machine is provided in Figure 5.6. Figure 5.6 Forming machine for chewable dosage forms, Source: Courtesy of Provisur. Critical quality attributes include appearance, assay, content uniformity, dissolution, impurities, and loss on drying. Capsules are a solid dosage form in which the drug is enclosed in a hard or soft soluble shell. The shells are usually made from a gelatin, cellulose, or polymeric material. The shells may be “hard”, consisting of two pieces (a body and a cap) that is typically filled with powder or beads. The hard capsules may be used as a mechanism for achieving extended drug release through such technologies as beads and osmotic pumps (Liu et al., 2014; Becker et al., 2014). Alternatively, the shell may be “soft”, consisting of a single piece (unit) that is typically filled with a solution or suspension containing the API. Soft capsules are often used for formulating poorly water-soluble drugs as a method of enhancing in vivo dissolution. Another advantage offered by the use of single unit capsules is that it avoids potential problems in achieving content uniformity. The potential for undesirable formulation effects need also be considered when selecting between hard or soft shell capsule formulations. In particular, because the contact between the shell wall and its liquid contents is more intimate than in dry-filled capsules, there is an increased risk of such interactions as gelatin crosslinking and pellicle (thin skin or film) formation. Quality attributes of capsules include assay, content uniformity, impurities, and dissolution. The API is mixed with other excipients to produce a final product for oral administration. Drug powders could be added in a solid form or feed, formulated as a soluble powder for addition to drinking water or milk replacer, or combined with emulsifying agents to facilitate their administration as liquid drenches. Challenges with powder dosage forms include undesirable taste attributes and potential problems with keeping denser powders from separating and concentrating at the bottom of the feed trough. Quality attributes include blend uniformity, impurities, and moisture. This dosage form consists of powder particles that have been granulated with other excipients to form larger particles, usually 2–4 mm in diameter. Granulation enhances ease of blending (e.g., with other excipients) and blend uniformity. Type A medicated article consists of an API with or without a carrier (e.g., calcium carbonate, rice hull, corn, gluten) and with or without inactive ingredients. The Type A medicated article may be used to manufacture another Type A, or a medicated article that has been diluted with additional feed (Type B or a Type C). The Type A medicated article is manufactured by mixing or granulating excipients to the API. The API can be synthesized chemically or produced by a fermentation reaction. Adding excipients enhance the uniformity of the mix, prevent segregation of the drug, and prevent segregation during transportation. Excipients can also be added to prevent dust formation during the mixing and to stabilize the drug in medicated feed. The FDA Guidance for Industry, #216 Chemistry, Manufacturing, and Controls (CMC) Information—Fermentation-Derived Intermediates, Drug Substances, and Related Drug Products for Veterinary Medicinal Use can be used as a general guide for the fermentation process used in biomass production. Biomass APIs are an unpurified fermentation product. The contents of the fermentation vessel are dried to produce the biomass drug substance. Characterization of an API typically addresses the active molecule and impurities, which could include: Controlled Internal Drug Release System (CIDR) is an intravaginal insert that is typically used in dairy cattle, goats, and sheep. The CIDR is a T-shaped device with flexible wings that collapse to form a rod which can be inserted into the vagina with an applicator. On the end opposite to the wings of the insert is a tail that is attached to facilitate removal of the device. The backbone of the CIDR is a nylon spine covered by a progesterone-impregnated silicone skin. The rate of progesterone release is influenced by the surface area of the implant and by the drug load. Upon its insertion, blood progesterone concentrations rise rapidly, with maximal concentrations reached within an hour. Progesterone systemic concentrations are maintained at a relatively constant level throughout the 7 days during which the insert is resident within the vagina. Upon its removal, systemic progesterone concentrations rapidly decline (Rathbone et al., 2002). The formulation and manufacture of injectable dosage forms involves a different set of considerations than that associated with oral products. The need for microbiological stability is the primary difference. Vials of injectable formulations may be concluded to be sterile upon release for marketing, but once the stopper is punctured, sterility can no longer be assured. Multiuse injection products must therefore be formulated with sufficient concentrations of bacteriostatic/fungistatic agents such as methylparaben to avoid growth of microorganisms in the remaining vial contents. As with solid pharmaceuticals, injections may be formulated as either IR or MR dosage forms. Since the in vivo residence time of injectable formulations are not constrained by GI transit times, there are few limitations on the duration of drug release from these dosage forms. To achieve the desired time release characteristics, formulators can manipulate product physical properties such as viscosity and particle size. One of the critical considerations when manufacturing parenteral dosage forms is the need to insure product sterility. Selection of an appropriate process necessitates an understanding of the potential chemical reactions that can occur. Accordingly, the strengths and limitations of the various sterilization processes depend on physical aspects unique to the type of pharmaceutical. In this regard, it is important to note that sterilization drives design of manufacturing processes, facilities, and formulation of sterile injections. Common sterilization techniques used for parenteral products include: Common types of injectable formulations include: Analytical testing of injection dosage forms may include the following parameters. Bacterial endotoxins: Gram-negative bacteria present during the manufacturing process release lipopolysaccharides, known as endotoxins, upon the death of the bacteria (Booth, 2001). Endotoxins are antigenic to most target species and cannot be completely eliminated by the most common sterilization techniques. USP Chapter <85> (USP, 2009b) describes tests to measure endotoxin levels (with results expressed either as Endotoxin Units (EU) or as International Units (IU)). Endotoxin limits for products administered by IM, SC, or IV routes historically have been based on the rabbit endotoxin sensitivity of about 5 EU/kg body weight divided by the dose of the injection per kilogram body weight (Rahman et al., 2014). Although it is recognized that each target animal may differ in its sensitivity to endotoxins, unique endotoxin limits have not been developed for individual species. Topical products may be formulated as solutions, suspensions, ointments, gels, creams, emulsions, or aerosol. Unlike transdermal formulations, these products are not intended to be systemically absorbed. The location of delivery can be the skin, the mammary gland, the eyes, or the ears. The location of the application dictates the appropriate excipients to insure that the API reaches and is retained within the site of action. One of the challenges encountered with these products is that systemic drug concentrations (e.g., blood level profiles) cannot be used to evaluate product performance. As a result, the formulation chemist needs to rely upon in vitro and physicochemical tests to evaluate the impact of formulation and manufacturing method on product CQAs. In turn, without extensive clinical trials and/or in situ model studies, allowable deviations in product physicochemical characteristics will be very difficult to define (Teng et al., 2009). Formulating the API so that it penetrates protective barriers such as skin can be a significant challenge. A number of complex systems have been developed to accomplish this goal including patches, ointments, suspensions, lotions, plasters, pastes, and dressings. Within this textbook, Chapters 2 and 47 discuss this topic further. The manufacturing process can have as great an influence on drug product quality and performance as the formulation. For that reason, the manufacturing process needs to be considered both from the perspective of the dosage form, batch size, and physicochemical properties of the drug and its excipients. As mentioned under our discussion of lubricants, something as simple as blending time or the order of excipient addition can make the difference between products that exhibit the desired in vivo characteristics versus those failing to exhibit the targeted quality attributes. The tablet manufacturing process can be classified as granulation (wet or dry granulation) or direct compression. Granulation is a process that causes an increase in particle size, thereby improving the flow, density, and compressibility of the powder (API and excipients). Granulation can be achieved by using a binder solution (wet granulation) or a dry granulation (without the addition of a binder solution). Wet granulation is the most commonly used method to manufacture veterinary tablets. Wet granulation (low shear, high shear, fluid bed, extrusion, and spheronization process of converting material into spheres) is a process of using a liquid binder (usually a hydrophilic colloid) to lightly agglomerate the powder mixture. This reduces the interparticulate friction and improves the fluidity and compressibility of the powder. The binder, which is distributed over large surface areas, acts as glue to overcome the lack of cohesiveness of the original API and the fillers. Wet granulation also improves the blend uniformity for soluble low-dosage drugs and is an effective technique to improve the dissolution rate of hydrophobic compounds. The quality of this technique is generally governed by the physicochemical properties of the powder and/or granulation from which tablets are composed. Wet granulation may not be suitable for an API that is sensitive to humidity or temperature. Dry granulation (roller compaction or slugging) is a process that creates granules by compaction of the powder blend under low pressures. This process often results in ribbons that are subsequently milled and screened to form granules of the desired particle size. The advantage of dry granulation is the absence of heat and moisture. However, it can lead to dust formation and may not be as efficient as wet granulation in terms of insuring the uniformity of the API content in the resulting granules. Direct compression is the tableting of ingredients in the absence of a preliminary granulation step. This process avoids many of the problems associated with wet and dry granulation and is best used for formulations that exhibit good flowability, compactibility, and compressibility. However, the product generated by direction compression methods is highly dependent on the intrinsic physical properties of the raw materials (especially fillers). Any variability in the quality of the raw materials may affect the flow and compression characteristics, causing the direct compression to be challenging. In an effort to overcome this hurdle, some excipients are now available to facilitate direct compression (e.g., fast flow lactose, dicalcium phosphate dibasic, microcrystalline cellulose). These excipients are manufactured consistently to assure their physical forms (e.g., particle size, particle shape), thereby facilitating improved formulation flowability and compressibility. Extrusion processes can be categorized as either ram extrusion or screw extrusion. Either technique is well suited for the precision extrusion of highly valuable materials. Process control monitoring includes zone temperature, the monitoring of torque, drive amperage, and pressure and melt viscosity. Temperatures are normally controlled by electrical heating bands and monitored by thermocouples. Recently, dry granulation using roller compaction has grown in popularity, as the process is economical, energy efficient, easily automated, and suitable for drugs that are sensitive to heat and moisture (Kona et al., 2014). In roller compaction, the powder blend is compressed and compacted between two counter-rotating rollers resulting in ribbons, which when milled produce granules of the desired size (Kostewicz et al., 2014). Following the use of roller compaction, the resulting granules are mixed with lubricant and/or other excipients and finally compressed into the tablets. Slugging is used to compress the formulation into relatively large tablets that are subsequently milled and screened to attain the desired size granules. The resulting granules are blended with lubricant and/or any other excipients and then finally compressed into tablets. The size and speed of tableting machines (presses) can vary, but their general operating principles are the same. Tableting processes typically use a powder blend or granulation. The powder blend or granulation is added to a hopper, which then flows in a controlled manner into dies. Above and below each die are punches that create the compression pressure necessary to form the tablet into the appropriate shape. Tablet shape is determined by the geometry of the die. Following compression, the newly formed tablets are ejected from the press and the process repeats. Upon examination of the attributes of the dosage forms described in this chapter, it is evident that the ability of the drug to dissolve in and diffuse through biological fluids is an essential component of product performance. The fundamental issue is developing an understanding of the physicochemical properties of the API and how to adjust the formulation so as to accommodate the critical factors influencing in vivo product performance. With respect to product dissolution, the critical factors can be identified in the Noyes–Whitney equation (Equation 5.9).
Principles of Pharmaceutics and Veterinary Dosage Forms
Introduction
Understanding the Active Pharmaceutical Ingredient
Physical Properties
Crystalline Form
Particle Geometry
Size:
Particle shape:
Dissociation Constants/pKa
Partition Coefficients
Log P:
Log D:
Melting Point
Rheology
Chemical Properties
Stability
Stereochemistry
Geometrical Configuration
API
S Form
R Form
Asparagine
(S) Bitter Taste
(R) Sweet Taste
Chloramphenicol
(S,S) Inactive
(R,R) Antimicrobial
Propranolol
(S) 100 × activity of (R)
Ethambutol
(S,S) Tuberculostatic
(R,R) Cause blindness
Etodolac
(S) Antiinflammatory
(R) Reduced activity
Verapamil
(S) Cardiovascular effect
(R) Cancer therapy
Optical Rotation
Solubility
Permeability
Formulation Elements
Excipients Typically Used in Veterinary Dosage Forms
Excipients for Solid Dosage Forms
Fillers (Diluents):
Binders:
Disintegrants:
Glidants:
Lubricants:
Coating agents:
Excipients for Modified Release Formulations
Matrix tablets:
Coated tablets
Excipients Typically Used in Liquid and Semisolid Formulations
Bulking agents:
Buffering agents:
Tonicity adjusting agents:
Preservatives:
Antimicrobial agents:
Chelating agents:
Antioxidants:
Solubilizing agents:
Complexing and dispersing agents:
Flocculating/suspending agents:
Wetting agents:
Thickening agents:
Coloring agents:
Humectants:
Flavors
Dosage Form Considerations
Types of Dosage Forms: Liquids and Semisolids
Solutions:
Suspensions:
Pastes:
Gels:
Lotions:
Cream:
Ointment:
Types of Dosage Forms: Solids
Tablets:
Immediate release (IR):
Modified release (MR):
Extended release (ER):
Delayed release:
Bolus:
Implants:
Chewable tablets:
Capsules:
Powders:
Granules:
Types of Dosage Forms: Medicated Feed
Types of Dosage Forms: Biomass Products
Types of Dosage Forms: Controlled Internal Drug Release System
Types of Dosage Forms: Liquid or Semisolid Dosage Forms
Parenteral products:
Types of Dosage Forms: Topical Dosage Forms
Types of Dosage Forms: Transdermal Drug Delivery
Manufacturing Process
Wet/dry granulation:
Direct compression:
Extrusion:
Roller compaction:
Slugging:
Tableting processes:
Factors Influencing Product Dissolution
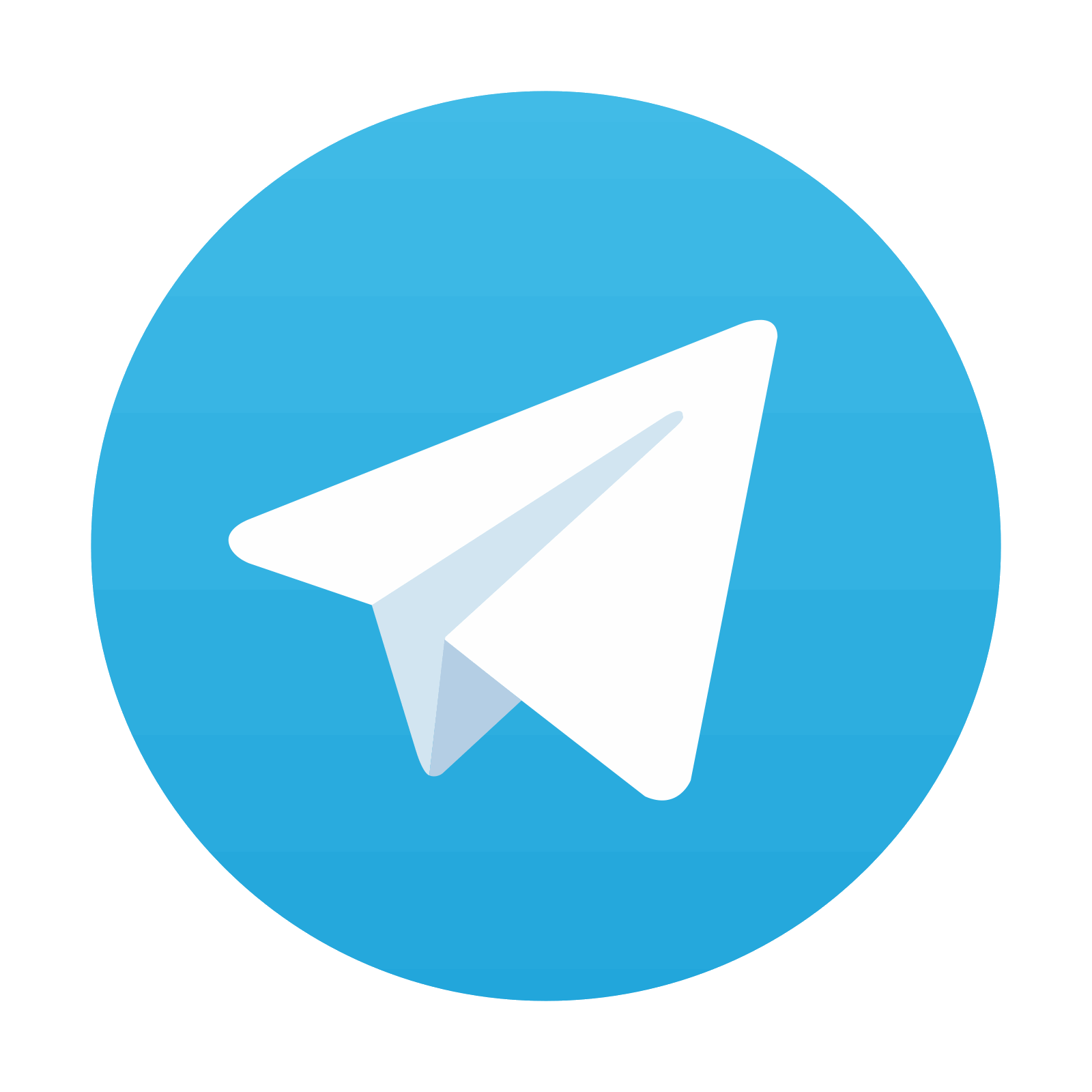
Stay updated, free articles. Join our Telegram channel
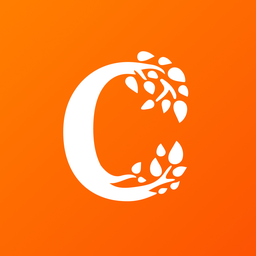
Full access? Get Clinical Tree
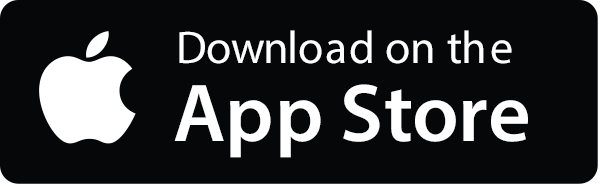
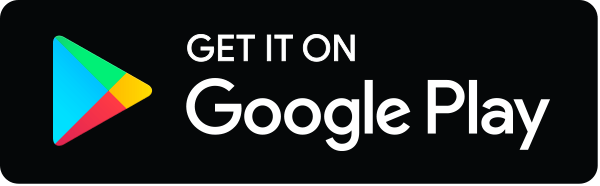