23 Maya M. Scott-Garrard, Melisa Rosenthal, and Deborah T. Kochevar The units of measure commonly utilized in discussion of fluid balance are presented in Table 23.1. Ions or electrolytes combine according to valence (charge) rather than molecular weight. Hence in the case of univalent ions, 1 mM = 1 mEq. One mM of a divalent ion provides 2 mEq. By expressing most electrolyte concentrations in milliequivalents per liter (mEq/l), and comparing the concentration of cations to anions in the body, it becomes clear that electroneutrality exists. Although extracellular cations are often more completely documented in the course of clinical investigations, anions, particularly chloride and bicarbonate, are the electrical counterbalance. Some electrolytes are measured in millimoles per liter (mM/l) because they exist in variable states of protein binding or valence. An example is total calcium, because protein binding confounds any simple assessment of ionized fraction. Phosphorus exists in variable proportions of phosphate and monohydrogen and dihydrogen phosphate, so no valence can be assigned and calculation of milliequivalence is therefore inaccurate. Since mEq/l are the most common and informative unit of comparison for most electrolytes, conversion formulas are also provided in Table 23.1 . Table 23.1 Units of measure and conversions commonly used in fluid therapy = mmol/l × valence = [(mg/dl × 10)/MW] × valence Solutes exert an osmotic effect in solution that is dependent only on the number of particles in solution, not on molecular weight or valence. Hence for nondissociable substances, 1 osmole contains 1 mole of substance. If a substance dissociates in solution, the number of osmoles is increased according to the number of particles generated per mole of dissociated substance. For example, each mmol of a completely dissociated NaCl solution yields 2 mOsm. Osmolarity refers to the number of osmoles per liter, and osmolality indicates the number of osmoles per kilogram of solvent (Rose, 1989). In physiological systems the difference between these two is usually small. The concept of osmolality explains why solutions of diverse chemical and electrical composition (e.g., 5% dextrose, 0.9% NaCl, and 1.3% sodium bicarbonate) can all be considered isotonic. For mammals, isotonic solutions equal approximately 300 mOsm. Semipermeable membranes separate most body compartments, allowing the free passage of water and selected solutes. The effective osmolality, or tonicity, of a solution is related to the ability of a solute to attract water and to sustain an increase in osmotic pressure as a result of water movement. For example, two substances with equal ability to attract water down a concentration gradient and across a semipermeable membrane may have very different effects on osmotic pressure, depending upon the movement of the substance itself through the semipermeable membrane. While the measured osmolality of a solution includes all osmoles, whether effective or ineffective, tonicity of a solution relates only to effective osmolality. For example, a solution containing 300 mOsm of nonpenetrating NaCl and 100 mOsm of urea, which can cross plasma membranes, would have a total osmolarity of 400 mOsm and would be hyperosmotic. However, if one put red blood cells in this solution, they would not shrink or swell, because the urea would diffuse into the cells and reach equilibrium inside and outside the cells. Thus, both extracellular and intracellular solutions would have the same osmolarity. There would be no difference in the water concentration across the membrane and no change in cell volume. The solution is therefore considered isotonic. Ultimately all fluids within the body are in dynamic equilibrium, but it is helpful during fluid therapy to consider body water as existing in several compartments since critical fluid shifts can and do occur. Determination of the volumes of these compartments is problematic, as can be deduced from the large number of different methods that have been used to estimate these volumes (Kohn and DiBartola, 1992). The most common method for assessment of volume in body fluid compartments depends upon intravenous administration of a known amount of a dye or radioisotope-tagged substance that distributes only in the compartment of interest. This is followed by assessment of dye or radioisotope concentration in the compartment. Ideally, the indicator substance must distribute rapidly and homogeneously, remain in the space to be measured, not be metabolized or bound, and be nontoxic. The volume of distribution (Vd) of a drug, or in this case a volume marker, may be derived according to the same principles of pharmacokinetics described in Chapter 3. Total body water (TBW) is approximated at 60% of body weight, but this figure varies from 50 to 75% depending upon age, lean body mass, and individual animal variations. Since fat is lower in water content than lean tissue, obesity is associated with decreased TBW (approximately 50%). To avoid overhydration of obese patients, fluid requirements are best estimated based on lean body mass. Very young animals are about 70–75% water, with TBW declining with advancing age. Table 23.2 provides estimates of selected volumes in dogs. TBW is broadly divided into two types: intracellular (ICF) and extracellular fluid (ECF). The ECF is further divided into four subcompartments: plasma volume, interstitial lymph fluid, transcellular fluid, and fluid present in dense connective tissue and bone. Table 23.3 provides experimentally derived blood volumes as percentages of body weight for various species. Table 23.2 Approximate volumes of selected fluid compartments in the dog. Source: Data from Kohn and DiBartola, 1992. Table 23.3 Approximate values for blood volumes of various animals expressed as percentages of body weight. Values represent averages from approximately 30 references. Transcellular fluid is found in diverse locations, including cerebrospinal fluid, pleural cavity, gastrointestinal tract, bladder, synovia, aqueous humor, and peritoneal cavity. Transcellular volumes vary greatly from monogastrics (1–6%) to horses and ruminants (10–15%), dependent largely upon the amount of fluid sequestered in the gastrointestinal tract. Transcellular volumes are not readily mobilized during volume deficits but are of importance in terms of drug disposition and equilibrium. In certain disease processes, transcellular fluids may accumulate, causing ascites, hydropericardium, hydrothorax, synovitis, or other conditions, depending on the location of fluid accumulation. Body solutes are not distributed homogeneously throughout TBW. Like drugs, every solute has a defined space or volume of distribution that can be assessed experimentally. As with estimation of body compartment volumes, determination of solute distribution is limited by the features of the labeled solute used. Because normal vascular endothelium is largely impermeable to formed blood elements and plasma protein, these cells and solutes are usually limited to the plasma. Vascular endothelium is freely permeable to ionic solutes, and the concentration of these ions is almost the same in interstitial as in plasma fluid. Table 23.4 provides estimations of ion composition in plasma of normal mammals. Table 23.4 Approximate average concentrations of cations and anions in plasma in normal mammals. Source: Gross, 1994. Reproduced with permission of Springer. aPhosphate exists in variable proportions of phosphate and monohydrogen and dihydrogen phosphate, so no valance can be identified and the number of mEq/l is therefore an estimate (Gross, 1994). The volume of ICF and ECF compartments is determined by the number of osmotically active particles in each space. ECF osmolality can be estimated from the following formula (Rose, 1989): Because cell membranes are permeable to urea and K+, these substances contribute only ineffective osmoles, as described earlier. At normal blood glucose concentrations, Na+ is the primary determinant of effective ECF osmolality. Because Na+ is the most abundant and osmotically active ECF cation, maintenance of an extracellular-to-intracellular sodium gradient is critical and is accomplished by the cell membrane Na+,K+-adenosine triphosphatase (ATPase) pump. This pump is also responsible for maintaining appropriate concentrations of intracellular K+. Because K+ is the most abundant intracellular cation, the ratio of intracellular-to-extracellular K+ concentration is the major determinant of the resting cell membrane potential (−70 to −90 mV). Because all body fluid spaces are isotonic with one another, the effective osmolality of the ICF, and indeed TBW, must be equal to that of the ECF. Acute addition or loss of fluid and/or solutes from the body inevitably results in alterations in compartment volumes and tonicity. Homeostatic shifts of fluid between compartments must then occur to return the system to isotonicity. The critical distribution of water between the plasma and the interstitium is maintained by the colloidal osmotic pressure of plasma protein (oncotic pressure). This is the force that draws water into the capillaries and balances the hydrostatic pressure driving water out. These so-called “Starling forces” describe the capillary balance between forces that favor filtration of water from plasma and those that retain vascular volume: where Kf represents permeability of the capillary wall, P represents hydrostatic pressure in the capillaries (Pcap) (blood) or tissues (Pif) (interstitial fluid), and π represents oncotic pressure generated by plasma protein (πp) or filtered proteins and glycosaminoglycans in the interstitium (πif). Applying Starling’s relationships yields the prediction that hypoproteinemia (decreased πp) will increase loss of vascular fluid and that water depletion (with a relative increase in πp and a decrease in Pcap) will promote reabsorption of interstitial fluid into the vasculature (Kohn and DiBartola, 1992). The volume of intracellular water in a given tissue is maintained by intracellular protein. As plasma water decreases, plasma protein competes with intracellular protein for water, resulting in cellular dehydration. Clinical alterations in plasma osmolality may be assessed by comparing measured osmolality in a patient to calculated serum osmolality as determined using Na+, K+, glucose, and BUN measurements (see the ECF osmolality equation provided above). Observed changes in the osmolal gap (difference between measured osmolality and the osmolality calculated from normal concentrations) may be useful in determining the presence of unmeasured osmoles associated with toxic substances such as ethylene glycol. The osmolal gap may also be useful in assessing shifts in plasma sodium concentration (Kohn and DiBartola, 1992). The number of cations in the ECF must equal the number of anions in order to maintain electroneutrality. In practice, only selected cations and anions are routinely measured in a clinical setting. Calculation of the difference between the commonly measured cations and anions in ECF yields the unmeasured anions, or anion gap (Oh and Carrol, 1977; Emmett and Narins, 1977). The anion gap calculation can be useful in assessing the etiology of metabolic acidosis and will be discussed in this context subsequently. Daily intake of water, nutrients, and minerals is normally balanced by daily excretion of these substances. Water turnover is the term used to describe input and output of body water over a given period of time. Values for water turnover, per 24 hours, in various domestic animals resting in cages or stalls range from about 40 to 132 ml/kg/day. The range is influenced by species, age, and physiological state (Adolph, 1939; Smith, 1970 unpublished data). Extremes of temperature, psychological state, disease, and other variables may change water demands markedly. Water turnover in mature dogs is approximated as 40–60 ml/kg/day, while immature and lactating animals may turn over approximately twice this amount (Muir and DiBartola, 1983). Maintenance fluid needs are defined as the volume of fluid required daily to maintain an animal in zero fluid balance, that is, no net gain or loss of water. Normal water intake occurs in response to thirst, which is stimulated by plasma hypertonicity and/or contracted ECF volume. Plasma hypertonicity, the primary stimulus, prompts osmoreceptors in the supraoptic and paraventricular nuclei of the hypothalamus to release vasopressin, also called antidiuretic hormone (ADH), which is released into the circulation at the level of the pituitary neurohypophysis. Binding of vasopressin to receptors in the distal nephron and renal collecting duct cells activates adenylyl cyclase and increases intracellular cyclic AMP. A protein kinase cascade initiated by activation of protein kinase A results in opening of luminal water pores in the tubule cell. Permeability of the collecting duct to water and reabsorption of water increase. Sustained release of vasopressin depends additionally upon calcium cycling across the plasma membrane and activation of protein kinase C–dependent pathways. Prostaglandins inhibit the renal response to vasopressin. Drugs with anticyclooxygenase activity that inhibit prostaglandin synthesis thereby enhance the action of endogenous vasopressin. Figure 23.1 summarizes the effects of selected drugs and electrolytes on vasopressin release and action. Figure 23.1 Effects of drugs and electrolytes on vasopressin release and mechanisms of cellular action. AA, arachidonic acid; AC, adenylyl cyclase; ATP, adenosine triphosphate; cAMP, cyclic adenosine monophosphate; PDE, phosphodiesterase; PGE, prostaglandin E. Source: Adapted from DiBartola 1992c. Reproduced with permission of Elsevier. If ECF volume and renal perfusion decrease, volume receptors in the renal juxtaglomerular apparatus respond, causing the secretion (or release) of renin, which converts angiotensinogen to angiotensin I. This is the rate-limiting step in the renin–angiotensin system. Angiotensin I is activated to the potent vasoconstrictor angiotensin II in the lung and in endothelial cells throughout the body by angiotensin-converting enzyme (ACE). Angiotensin II stimulates the zona glomerulosa of the adrenal cortex to secrete aldosterone, which, in turn, causes increased reabsorption of sodium from the distal nephron with excretion of K+ and H+. Due to the increased concentration of sodium, plasma becomes hypertonic, causing vasopressin release and water retention. Water intake occurs in response not only to thirst but also to hunger. Water content of food may be as low as 10% (dry food) or as high as 90% (succulent green pasture). Canned pet foods generally contain more than 70% water, and semimoist foods are intermediate (20–40% water) (Lewis and Morris, 1987). Intake of dietary water is governed centrally by appetite control mechanisms rather than by fluid and electrolyte homeostasis. In addition to water intake related to eating and drinking, metabolic water is produced endogenously by catabolism of proteins, fats, and carbohydrates (approximately 5 ml/kg/day) and represents about 10–15% of total water intake in dogs and cats (Anderson, 1983). Normal water loss occurs via urine, fecal water, and saliva (sensible loss), with insensible losses occurring via evaporation from cutaneous and respiratory epithelia. Insensible losses account for TBW elimination of about 15–30 ml/kg/day in healthy, sedentary animals in a thermoneutral environment (Kohn and DiBartola, 1992). Metabolic rate, and therefore a portion of daily water turnover, are directly proportional to the ratio of body surface area to total volume. For example, the surface area to volume ratio in a puppy is much larger than in an adult dog and the puppy has a higher basal metabolic rate. Both lead to a much greater evaporative loss of water from the skin per unit volume. Hence, daily water turnover per unit body weight may be nearly twice that of the adult animal. Small, immature animals are therefore at greater risk for insensible water loss than large, mature animals. The most important and predictable loss of water in healthy, sedentary animals, in a thermoneutral environment, occurs via the urine. Urinary losses can vary from 2 to 20 ml/kg/day. Daily urinary water losses may be divided into obligatory water loss and free water loss (Kohn and DiBartola, 1992). Obligatory water loss represents water eliminated in order to excrete the daily renal solute load. The renal solute load is derived from dietary sources of protein and minerals and consists of urea, Na+, K+, Ca++, Mg++, NH4+, and other cations, and PO43−, Cl−, SO42−, and other anions. Hence, daily renal solute load is a function of the quantity and composition of food ingested. Urea accounts for two-thirds of the urinary solute load in dogs (O’Connor and Potts, 1969). In normal animals increased urine solute load is eliminated by an increase in urine volume (obligatory water loss) rather than a marked increase in urine osmolality. Hence, urine osmolality is not generally maximized in order to accomplish steady-state elimination of solutes. Obligatory renal water loss is clinically important for removal of renal solutes but also because this type of water loss will continue even in states of relative water deficit. Free water loss represents water excreted unaccompanied by solute. Excretion of free water is controlled by vasopressin and increases during relative water excess or hypotonicity and decreases during water deficit or hypertonicity. Obligatory fecal water loss occurs in order to excrete fecal solutes. Fecal losses ordinarily account for 2–5% of TBW losses and vary with the species. Feces typically contain 50–80% water (Kohn and DiBartola, 1992). Elimination or conservation of body water and solutes via the kidneys depends upon the processes of glomerular filtration and renal tubular reabsorption and secretion. A major mechanism for conservation of water is urine concentration. The canine kidney can concentrate urine to as much as 2400 mOsm, compared to 1200–1400 mOsm achieved in human urine. Elimination of substances via the urine depends upon renal clearance of each substance from the plasma. The volume of plasma that must be filtered each minute to account for the amount of substance appearing in the urine each minute under steady-state conditions defines renal clearance of that substance. As much as 20% of cardiac output is directed to the kidneys, with blood entering a renal glomerulus through an afferent arteriole and leaving through an efferent arteriole. Resistance changes in afferent and efferent capillaries regulate glomerular filtration rate (GFR). For discussions of normal and abnormal renal physiological function the reader is referred to any standard physiology text. An understanding of the complexities of renal function is crucial to the understanding of water, acid–base, and electrolyte balances. As glomerular filtrate flows through the tubules, most of the water (greater than 90%) and varying amounts of solute are reabsorbed into the peritubular capillaries. The composition of the tubular reabsorbate closely approximates that of ECF. Reabsorption is largely achieved by transport of electrolytes and other solutes in two steps: (i) absorption of solutes from tubular fluid into tubular cells and (ii) movement of solutes from tubular cells into the ECF. Several types of transport account for tubular reabsorption of solutes, including passive transport (simple diffusion), facilitated diffusion, active transport, and cotransport. These mechanisms are discussed in more detail in the context of diuretic drugs (Chapter 24) and are summarized in Figure 23.2, which depicts some of the functional processes for regulation of salt and water transport in different segments of the nephron. Figure 23.2 Functional processes for regulation of salt and water transport in a nephron. Source: Thier 1987. Reproduced with permission of Taylor & Francis. As much as 60–65% of filtered solute is reabsorbed in the proximal tubule accompanied by osmotically proportional amounts of water. The tubular fluid at the distal portion of the proximal tubule becomes slightly hypoosmotic. Passive reabsorption of substances, especially sodium and chloride, continues in the thin segment of the loop of Henle. The thick ascending limb of the loop of Henle and the distal convoluted tubule are relatively impermeable to water but actively reabsorb solute. Sodium and chloride enter tubular cells in the thick ascending limb of Henle’s loop by crossing the luminal membrane coupled to potassium in a proportion of 1 Na+ : 1 K+ : 2 Cl−. Sodium is then actively extruded across the basolateral membrane to maintain intracellular sodium at low levels. Potassium and chloride leave the tubular cell passively. Two consequences of this are decreased concentration of sodium and chloride in the tubular lumen and increased concentration of each in interstitial fluid. A concentration gradient across the tubular epithelium is established, and this becomes multiplied in a longitudinal direction by the countercurrent mechanism. The collecting ducts are responsive to vasopressin, and in its presence the ducts become highly permeable to water. Tubular fluid equilibrates with hyperosmotic interstitium, and hypertonic (concentrated) urine results. In the absence of vasopressin, the ducts are relatively impermeable to water. In this case, sodium and chloride have been reabsorbed proximally to the collecting ducts, tubular fluid is hypoosmotic, and voided urine is dilute (Thier, 1987). Renal reabsorption of sodium in the distal nephron is increased by aldosterone, a mineralocorticoid synthesized in the zona glomerulosa of the adrenal cortex. Aldosterone is produced and released in response to stimulation by angiotensin II, hyperkalemia, and by a decrease in dietary sodium intake. Adrenocorticotropic hormone (ACTH) and hyponatremia play permissive roles in promoting aldosterone secretion. Increased dietary sodium and atrial natriuretic peptide (ANP) decrease aldosterone production. ANP is a polypeptide released from atrial and ventricular myocytes in response to atrial distention associated with volume expansion. ANP causes vascular smooth muscle relaxation, inhibits production of aldosterone in the adrenal glands, and blocks the production of angiotensin II. Study results suggest that parathyroid hormone (PTH) is required for augmented ANP secretion in response to acute volume loading in rats. PTH may play an important role in the regulation of fluid homeostasis via control of ANP (Geiger et al., 1992). In general, chloride is reabsorbed with sodium throughout the nephron. As previously noted, chloride is exchanged in a ratio of 1 Na+ : 1 K+ : 2 Cl− in the thick ascending limb of Henle’s loop during sodium reabsorption. Because the cotransporter in this exchange has a very high affinity for both Na+ and K+, luminal Cl− concentration is normally the rate-limiting step in NaCl entry into the cell (Gregor and Velazquez, 1987). Additional active and passive processes contribute to proximal Cl− reabsorption in the renal tubules. Chloride exchange for formate appears to occur via an anion exchanger in the luminal membrane. Low concentrations of filtered formate combine with H+ to form formic acid (HF) in the tubular lumen. Because HF is uncharged, it moves freely into the tubular cell. Two additional mechanisms set the stage for conversion of HF back to formate and H+. First, basolateral Na+,K+-ATPase pumps maintain a low intracellular sodium concentration, and this, in turn, allows for the continued exchange of Na+-H+ across the luminal membrane. As Na+ is reabsorbed and H+ is secreted, the interior of the cell is left with a lower [H+] than the tubular lumen. Under these conditions HF is converted back to H+ and formate, providing for continued chloride–formate exchange. Reabsorbed chloride is returned to the ECF across the basolateral membrane by selective Cl− channels and a K+-Cl− cotransporter (Rose, 1994). Additional transport mechanisms in type B intercalated cells in the cortical collecting tubule may exchange bicarbonate for chloride. The favorable inward concentration gradient for chloride (lumen concentration greater than inside the cell) presumably provides the energy for bicarbonate secretion via this mechanism (Bastani et al., 1991). Understanding the mechanisms for renal regulation of acid–base balance and electrolyte transport is increasingly dependent upon use of transgenic mice in which the function and regulation of key transporter proteins can be assessed (Cantone et al., 2006). Dehydration may be considered in three general categories: hypertonic, isotonic, and hypotonic. Pure water loss and loss of hypotonic fluid lead to hypertonic dehydration. As pure water is lost from the ECF, fluid shifts from the intracellular to the extracellular compartment in response to increased osmolality. The resulting proportionate distribution of volume loss results in fewer clinically detectable signs of volume depletion in the patient. Causes of dehydration associated with pure water deficit include hypodypsia due to neurological disease, diabetes insipidus, respiratory losses during exposure to elevated temperatures, fever, and inadequate access to water. Loss of hypotonic fluid, as compared to pure water, results in a greater depletion of ECF volume since there is less osmotic drive to pull volume from the intracellular space. Hypotonic fluid losses are common and have been subclassified as extrarenal and renal. Extrarenal losses could include gastrointestinal (e.g., vomiting or diarrhea) or third-space loss (e.g., pancreatitis, peritonitis, as a result of surgery or cutaneous injury). Third spacing is a term used to describe extravasation of fluid from the vascular compartment into extravascular spaces. As tonicity of lost fluid approaches or exceeds normal plasma osmolality (about 300 mOsm/kg), disproportionate depletion of ECF causes more evident clinical signs of dehydration. Volume depletion would likely be the most clinically apparent in cases of hypertonic fluid loss. Estimations of percent dehydration based on clinical signs are given in Table 23.5. Skin elasticity is a useful indicator of hydration status. However, age of the animal, body condition, and the technique used for evaluating elasticity may affect hydration assessment. With advancing age or cachexia, loss of fat and protein may account for decreased skin elasticity unrelated to hydration. Conversely, obese animals are likely to retain skin elasticity longer in the face of dehydration. Possibly as a result of variations in elastin content of skin, some species display smaller changes in elasticity for a given degree of dehydration. This may be clinically important in the horse. While dry mucous membranes can indicate dehydration, open-mouthed breathing associated with respiratory disease may cause misleading mucous membrane dryness. Degree of enopthalmos is considered a very useful parameter in assessment of dehydration in large animals. For example, the measured gap between the eyeball and orbit has been included as a guideline for assessment of dehydration in neonatal calves. A gap less than 0.5 cm is correlated with 9–10% dehydration, and a gap greater than 0.5 cm suggests 11–12% loss of hydration (Naylor, 1996). A study in diarrheic calves evaluated several clinical and laboratory parameters to determine which were most useful in assessment of dehydration. Factors assessed included extent of enophthalmos, skin-tent duration on neck, thorax, and upper and lower eyelids, heart rate, mean central venous pressure, peripheral (extremities) and core temperatures, packed-cell volume, and hemoglobin and plasma protein concentration. The best predictors of degree of dehydration were extent of enophthalmos, skin elasticity on neck and thorax, and plasma protein concentration (Constable et al., 1998). Laboratory parameters such as hematocrit, plasma protein, and osmolality are often useful, but assessment should include consideration of possible preexisting derangements, such as anemia or hypoproteinemia, that could confound interpretation. If an accurate previous body weight is known, serial changes in weight are considered a very useful and accurate measurement in determining degree of dehydration. Table 23.5 Physical findings in dehydration As the most important and abundant ECF cation, sodium is essential for proper maintenance of membrane potentials, initiation of action potentials, and, according to strong ion difference theory, maintenance of acid–base balance. Plasma sodium concentration and plasma osmolality generally vary in parallel since sodium and its associated anions account for greater than 95% of plasma osmolality. Plasma sodium concentration reflects the ratio of body sodium ion concentration to TBW. Total body sodium content, however, is independent of plasma sodium concentration and may be increased, decreased, or unchanged in the presence of hyper- or hyponatremia. Clinical signs associated with alterations in serum sodium are more related to the rapidity of change rather than to the magnitude of sodium increase or decrease. Hypernatremia (e.g., >155 mEq sodium/l in dogs) and ECF hypertonicity can be caused by a loss of pure water, a loss of hypotonic fluid (extrarenal or renal), or a gain of impermeable sodium-containing solute (Figure 23.3). Clinical signs of hypernatremia are usually observed in dogs and cats as sodium concentration approaches and exceeds 170 mEq/ml. The signs seen are related to the osmotic movement of water out of cells. Negative effects of cellular dehydration are most pronounced in the brain and lead to the characteristic neurological deficits associated with hypernatremia. These deficits include abnormal behavior and mentation, ataxia, seizures, and coma. The more rapidly water shifts out of brain cells, the greater the chance that decreased brain volume will lead to rupture of cerebral vessels and focal hemorrhage (Arieff and Guisado, 1976). If sodium concentration or concentration of sodium-containing impermeable solute increases slowly, the brain attempts to adapt to the hypertonic state by production of intracellular solutes (e.g., sugars, amino acids) known as “idiogenic” osmoles. Production of these osmotically active substances protects the cell by retaining intracellular volume and preventing cellular dehydration. In addition to neurological deficits, other clinical signs of hypernatremia include thirst, anorexia, lethargy, vomiting, and muscle weakness. If hypernatremia is related to hypotonic fluid loss, then clinical signs of dehydration (as previously described) may be present. If a gain of sodium has caused the hypernatremia, volume overload may be a problem, especially in patients with cardiac disease. Figure 23.3 Summary of classification, causes, and treatment of hypernatremia. See text for additional details of treatment. Restoration of ECF volume and tonicity is of primary importance in treatment of hypernatremia. Volume replacement must be accomplished slowly to avoid rapid shifts in plasma osmolality. In general, the rate of fluid administration is determined by the rate of onset of the hypernatremia. When treating chronic hypernatremia, the serum sodium concentration should drop at a rate that does not exceed 0.7 mEq/l/h (O’Brien, 1995). If plasma osmolality drops quickly, water may be attracted intracellularly by idiogenic osmoles, resulting in development of cerebral edema. Patients with clinical hypernatremia are also often dehydrated, and need volume resuscitation. Custom fluid combinations can be designed to gradually bring down sodium while being able to give high rates of fluids to replace volume. In the case of pure-water loss, volume can be replaced with 5% dextrose in water over a 48- to 72-hour period. Since the dextrose ultimately enters cells and is metabolized, 5% dextrose administration is essentially replacement with pure water. Use of a 1 : 1 mixture of normal saline with 5% dextrose solution yields an isotonic solution of 2.5% dextrose, 0.45% saline that has also been utilized. This solution decreases plasma tonicity more slowly and decreases the chance for cerebral edema. Hypotonic fluid losses should generally be replaced with an isotonic crystalloid solution. If hypernatremia has resulted from addition of sodium or sodium-containing impermeable solute, then administration of 5% dextrose and water should be accomplished cautiously to avoid pulmonary edema. Diuretics may be useful in promoting saluresis (sodium excretion) as ECF volume is restored (Marks, 1998). Evaluation and treatment of hypernatremia in critically ill cats was reviewed with emphasis on the importance of careful monitoring and early recognition of signs for positive therapeutic outcomes (Temol et al., 2004). Causes of hyponatremia (<135–140 mEq sodium/l) are best categorized if two additional variables, osmolality and hydration, are also considered. As indicated in Figure 23.4, the more common causes of hyponatremia are accompanied by decreased plasma osmolality (<290 mOsm/kg) with or without volume depletion. If volume depletion exists with hyponatremia, then loss of body sodium has exceeded water loss. Physiological responses to hypovolemia lead to impaired water excretion and a relative dilution of the sodium remaining in body fluids. Hypovolemia causes decreased renal perfusion and GFR, leading to a decline in water excretion. Slower movement of filtrate through renal tubules enhances isosmotic reabsorption of salt and water in the proximal tubules and decreases presentation of tubular fluid at distal diluting sites. Additionally, hypovolemia prompts vasopressin release, further impairing water elimination. Finally, thirst related to hypovolemia results in consumption of low-sodium fluids that also dilute existing plasma sodium (DiBartola, 1992c). Figure 23.4 Summary of classification, causes, and treatment of hyponatremia. See text for additional details of treatment. Hyponatremia accompanied by hypervolemia and low plasma osmolality occurs in clinical disorders where there is a physiological perception of volume depletion by in vivo volume detectors. The physiological response is volume expansion. For example, in congestive heart failure, decreased cardiac output is sensed as volume depletion by baroreceptors. Release of vasopressin impairs water excretion, leading to expanded vascular volume. Decreased effective circulating volume and decreased renal perfusion also lead to activation of the renin–angiotensin–aldosterone system. Enhanced renal retention of sodium contributes to expanded vascular volume. In cirrhosis and the nephrotic syndrome, hypoalbuminemia and decreased oncotic pressure may contribute to decreased effective circulating volume and, ultimately, vasopressin release and volume expansion. Other features of hepatic and renal disease also contribute to decreased circulating volume and/or impaired water excretion (DiBartola, 1992c). Hyponatremia is relatively less common when associated with increased plasma osmolality. The most frequent cause of sodium decreases in the presence of increased plasma osmolality is the increased circulating glucose levels associated with diabetes mellitus. Each 100 mg/dl increase in glucose results in a measured decrease of serum sodium by 1.6 mEq/l (Katz, 1973). In response to the increased concentration of serum glucose, water shifts from the intracellular to the extracellular compartment, resulting in dilution of measured sodium. Serum osmolality remains high due to elevated glucose concentrations. Hyponatremia associated with normal plasma osmolality is referred to as pseudohyponatremia. The decreased sodium concentrations are spurious and are almost universally related to technical difficulties in sodium measurement when plasma lipid or protein concentrations are high. As with hypernatremia, clinical signs of hyponatremia are more severe if sodium concentration changes rapidly than if it changes over a more prolonged period of time. If sodium concentrations and plasma osmolality decrease quickly, water shifts out of the ECF and into cells. The central nervous system (CNS) is most affected by a rapid fluid shift, which, in hyponatremia, results in development of cerebral edema. If onset of hyponatremia is slow, the brain can adjust cell volume by decreasing intracellular osmolality and preventing influx of water from the ECF. Patients with chronic hyponatremia will also adjust intracellular osmolality to an extent that clinical signs may not be obvious even though sodium concentrations are quite low. Treatment of hyponatremia varies with etiology of the disorder. The goals of therapy are to manage the underlying disease and, if necessary, to increase serum sodium and osmolality. Infusion with conventional crystalloid solutions (e.g., normal saline or lactated Ringer’s solution) is reported to accomplish sodium and volume replacement in hyponatremic, hypovolemic patients (DiBartola, 1992c). Use of hypertonic saline solutions is not recommended since overly rapid correction of hyponatremia may do more harm than good. Chronic hyponatremia, in which the brain has adjusted to the decrease in osmolality and sodium, must be handled cautiously to avoid brain dehydration and injury, including osmotic demyelination syndrome. This syndrome, often occurring several days after correction of hyponatremia, results from areas of demyelination caused by treatment-induced increases in serum sodium concentration. Dogs with asymptomatic chronic hyponatremia are best treated by mild water restriction and monitoring of serum sodium. Chronic, symptomatic dogs should be treated such that the rate of increase of serum sodium does not exceed 10–12 mEq/l/day (0.5 mEq/l/h) (DiBartola, 1998). Again, the most important therapeutic goal in management of hyponatremia should be treatment of the underlying disease. Fluid loss associated with small bowel diarrhea often results in greater loss of HCO3− than chloride due to loss of alkaline pancreatic secretions and bile and HCO3− secretion in exchange for Cl− in the ileum. The resulting hyperchloremic metabolic acidosis is characterized by a normal anion gap. Additional causes and treatment for hyperchloremic metabolic acidosis will be considered subsequently under the heading of metabolic acidosis. Please refer to the discussion of hypernatremia for treatment of hyperchloremia associated with loss of free water. Hypochloremia may be seen in patients with fluid losses due to vomiting or excessive diuretic administration. Hypochloremic metabolic alkalosis may develop in these cases because an excess of chloride is lost, leading to decreased filtered Cl− in the renal tubules. As previously noted, activity of the Na+-K+-2Cl− cotransporter in the luminal membrane of the macula densa cell is primarily determined by the availability of Cl−. In hypochloremia, less Cl− is delivered, resulting in less NaCl reabsorption, promotion of renin release leading to secondary hyperaldosteronism, and increased distal H+ secretion. If further Na+ reabsorption does occur, then Na+ must be accompanied by an anion other than chloride, usually bicarbonate, or must be exchanged for a secreted cation, either H+ or K+. In addition, bicarbonate secretion in exchange for chloride, which is thought to occur in intercalated cells of the cortical collecting tubule, will decrease since this process is presumably driven by a favorable inward gradient for Cl−. As luminal [Cl−] decreases, the gradient is dissipated and bicarbonate is retained in the system. All of the foregoing mechanisms promote retention of base and excretion of H+, leading to a hypochloremic metabolic alkalosis (Rose, 1994). Treatment with chloride-replete fluid such as normal saline is usually adequate to resolve chloride-responsive alkalosis. Potassium depletion may also promote a metabolic alkalosis and should be addressed as needed by addition of potassium chloride to fluids. As the major intracellular cation, potassium concentrations inside (145 mEq/l) and outside (3.5–5.5 mEq/l) the cell are maintained by the Na+,K+-ATPase pump. Under normal circumstances each pump actively transports three sodium ions out of and two potassium ions into the cell, but the ratio can change depending upon the circumstances. The ratio of intra- to extracellular concentration of potassium ([K+]i/[K+]o) is the major determinant of resting membrane potential. Resting membrane potential is crucial to normal membrane excitability associated with cardiac conduction, muscle contraction, and nerve impulse transmission. The normal dietary intake of potassium is much more than the body requires. About 90% of this intake is excreted in the urine, with the remainder of what is not required eliminated in the stool. Plasma potassium concentration is determined by the movement of potassium into or out of cells. Two important factors stimulating the transport of potassium into cells are insulin and β-adrenergic stimulation (Clausen and Flatman, 1987). Aldosterone is the primary determinant of potassium secretion across renal tubular epithelial surfaces. Most filtered potassium (60–80%) is reabsorbed in the proximal tubule. In the early proximal tubule, potassium enters the tubular cell at the luminal surface by active transport. The intracellular concentration of potassium is high, and the lumen of the tubule is negatively charged relative to the interior of the early proximal tubular cell. Potassium passively exits the basolateral membrane of the tubular cell down a favorable chemical concentration gradient. In the mid-to-late proximal tubule, the tubular lumen is relatively more positively charged than the tubular cell interior. This favors the passive reabsorption of potassium. Potassium again exits on the basolateral side of the tubular cell down a concentration gradient. Potassium reabsorption by intercalated cells in the distal nephron is similar to the process in the early proximal tubule and involves active transport at the luminal cell membrane followed by passive diffusion from the cell at the basolateral membrane. Tubular secretion of potassium is aldosterone mediated and occurs in the distal nephron (late distal tubule or connecting tubule of the collecting duct system) primarily in the “principal” cells of the collecting tubules. Additional information on mechanisms of collecting duct system reabsorption and secretion is given in Chapter 24 (Figure 24.2). Principal cells are rich in Na+,K+-ATPase and respond to aldosterone by increasing the number and activity of Na+,K+-ATPase pumps in the basolateral membrane. The increasing luminal membrane permeability to sodium causes greater lumen negativity relative to the tubular cell interior and increases luminal permeability to potassium. This facilitates potassium secretion into the tubule lumen. Aldosterone-stimulated Na+,K+-ATPase actively pumps potassium out of the peritubular fluid through the basolateral tubular cell membrane. Movement of potassium from the tubular cell through the luminal membrane and into the tubule lumen is favored by relative negativity of the lumen compared to the interior of the distal tubule cell (Black, 1993). When plasma potassium concentration is low, secretion of potassium by the principal cells is reduced while hydrogen ion secretion may be increased. Active potassium reabsorption by intercalated cells in the distal nephron is also stimulated by a potassium deficit. An additional factor affecting the movement of potassium across tubular cells is related to tubular flow rate. A rapid flow of filtrate through the tubules maximizes the potassium concentration gradient between the tubular cell interior and the lumen of the tubule and enhances potassium excretion. A reduction of tubular flow slows secretion by allowing a relatively greater concentration of potassium to be maintained in the lumen of the distal tubule (DiBartola and Autran de Morais, 1992). Disorders of potassium balance have marked effects on excitable membranes. The difference between the resting membrane potential and the membrane potential required for depolarization (threshold potential) determines the excitability of a cell. Hypokalemia makes the resting membrane potential more negative, thereby hyperpolarizing the cell and increasing the difference between resting and threshold potentials. Hyperkalemia causes the resting membrane potential to become more positive, hypopolarizing the cell and causing hyperexcitability. In hyperkalemia, if the resting potential decreases to less than the threshold potential, the cell depolarizes but is incapable of repolarizing, resulting in loss of cell excitability (DiBartola and Autran de Morais, 1992). In cardiac muscle this results in diastolic arrest; in vascular smooth muscle hyperkalemia causes vasoconstriction. Changes in pH affect the distribution of potassium between the ICF and the ECF. When acidosis is present, potassium moves out of cells in exchange for hydrogen, which moves intracellularly. In the distal tubule more hydrogen, and relatively less potassium, may be exchanged for sodium at the luminal membrane, leading to decreased potassium excretion. Based on these general principles, a clinical rule of thumb predicts that each 0.1 unit decrease in pH will be accompanied by a 0.6 mEq/l increase in serum potassium concentration. Conversely, in alkalosis potassium tends to move into cells in exchange for extracellular movement of hydrogen. Hypokalemia has been thought to promote alkalosis because less potassium is available to be exchanged for sodium in the distal tubule. Instead, sodium exchanges for hydrogen at the luminal membrane, leading ultimately to reclamation of bicarbonate and increased systemic pH. At the same time that systemic pH is increasing, secreted hydrogen ions exchanged for sodium cause the urine pH to decline. Although the principles outlined above are commonly stated and widely applied clinically, it is not clear that these explanations are adequate. In acidosis, the effect of pH changes on potassium translocation varies with the nature of the acid anion, blood pH and HCO3− concentration, osmolality, hormonal activity, and liver and renal function (DiBartola and Autran de Morais, 1992). Although changes in serum potassium have been documented during acute mineral acidosis caused by HCl or NH4Cl (Adrogue and Madias, 1981), acute metabolic acidosis caused by organic acids did not increase serum potassium as predicted (Oster et al., 1980; Adrogue and Madias, 1981). In certain conditions (e.g., diabetic ketoacidosis), hyperkalemia may be more directly associated with hyperosmolality and insulin deficiency than with the acidosis itself. In lactic acidosis, increased serum potassium concentration may be the result of release of intracellular potassium caused by cell breakdown associated with decreased peripheral perfusion (Black, 1993). Metabolic acidosis associated with both mineral and organic acids may directly or indirectly stimulate aldosterone secretion. The effects of aldosterone facilitate excretion of the acid load and, presumably, potassium, although one study failed to show any changes in serum potassium concentration (Perez et al., 1980). Early studies of the effects of hypokalemia on acid–base balance may have overlooked the key role of chloride depletion in causing metabolic alkalosis (DiBartola and Autran de Morais, 1992). When pure potassium depletion is created iatrogenically in rats, metabolic alkalosis results. However, in dogs, potassium deficit with normal chloride levels leads to metabolic acidosis due, presumably, to a distal renal tubular acidification defect (Garella et al., 1979). Total body potassium may be normal, decreased, or increased with hyperkalemia. Clinical signs of hyperkalemia (>7.5 mEq/l) are generally associated with changes in membrane excitability and are more severe if the increase in potassium has been rapid. Muscle weakness, twitching, and irritability may occur. Electrocardiographically determined cardiac effects may include extrasystoles, intraventricular conduction blocks, high-peaked T waves, altered QT interval, widened QRS interval, decreased amplitude or disappearance of P waves, depressed ST segment, ventricular asystole, or fibrillation. Causes of hyperkalemia are summarized in Table 23.6. The more common causes are related to decreased urinary potassium excretion. Pseudohyperkalemia related to hemolysis can occur in species that have high red cell potassium concentrations similar to humans. Dogs, sheep, and cattle can be divided into two groups based on Na+,K+-ATPase activity in red cell membranes. Those animals with high activity and high intracellular potassium concentrations are at risk for hyperkalemia caused by hemolysis. Animals with genetically determined low activity and low intracellular concentrations of potassium are unlikely to suffer from pseudohyperkalemia since the concentration of potassium in red cells resembles the concentration in the ECF (DiBartola and Autran de Morais, 1992). Table 23.6 Causes of hyperkalemia. Source: Adapted from DiBartola, 1992a. Reproduced with permission of Elsevier. The effects of several different drugs may impact serum potassium concentration. Since potassium uptake by cells is mediated in part by catecholamines at β receptors, beta blockers decrease intracellular potassium movement and increase ECF potassium concentrations. Angiotensin-converting enzyme (ACE) inhibitors may cause hyperkalemia by interfering with angiotensin II–mediated aldosterone secretion. Prostaglandin inhibitors, heparin, and selected potassium-sparing diuretics (e.g., spironolactone) increase serum potassium by decreasing the secretion of aldosterone or by blocking its activity. In many cases drugs alone may not have a marked effect on serum potassium concentration but if combined with a potassium load or decreased renal function may cause clinically significant hyperkalemia. Treatment of hyperkalemia varies with the severity of the condition in terms of magnitude and rapidity of onset. Emergency treatment is indicated if potassium rises quickly and exceeds 6.0–8.0 mEq/l (Phillips and Polzin, 1998). Serum potassium concentrations less than these do not typically induce life-threatening cardiotoxicity and can usually be managed with administration of potassium-free fluids. More aggressive treatment is necessary if electrocardiographic signs suggest toxicity. Additional measures that may be taken in treatment of severe hyperkalemia are summarized in Table 23.7. Some are directed toward increasing movement of potassium from the extracellular to the intracellular compartment (i.e., glucose, insulin, and sodium bicarbonate), while others are intended to decrease potassium from the ECF by enhanced renal excretion (e.g., diuretics) or decreased gastrointestinal absorption (i.e., orally administered potassium-binding resins such as sodium polystyrene sulfonate). Table 23.7 Therapeutic considerations in the management of severe hyperkalemia Therapy with calcium gluconate is included as part of the emergency treatment of hyperkalemia because changes in membrane excitability associated with alterations in potassium may be exacerbated by abnormalities in ionized calcium. Ionized calcium affects the threshold potential of a membrane and, when calcium is decreased, brings threshold closer to resting membrane potential, resulting in greater membrane excitability. An increase in ionized calcium has an opposing effect on membrane excitability by increasing the threshold potential and making depolarization more difficult. Hence, hypocalcemia exacerbates hyperkalemia while hypercalcemia counteracts hyperkalemia. Since 97% of total body potassium is intracellular, depletion can occur with no change in plasma potassium concentration or even with an increase if acidosis is present. Clinical signs of hypokalemia (<2.5–3.0 mEq/l) can include weakness of skeletal and respiratory muscles and loss of intestinal smooth muscle tone. As in hyperkalemia, cardiac changes occur as potassium concentration changes. Supraventricular and ventricular arrhythmias are most commonly observed in animals. ECG hallmarks of hypokalemia in humans are flattened or inverted T waves, depressed S-T segment, and the appearance of U waves. Prolongation of the QT interval and U waves have been reported in dogs but are not as consistently seen as they are in humans. Hypokalemia is increasingly recognized as an important clinical problem in cats, especially in association with chronic renal failure and geriatric animals (Phillips and Polzin, 1998). Feline hypokalemic polymyopathy syndrome, characterized by generalized muscle weakness associated with hypokalemia, is often manifest in cats as ventroflexion of the head and a stiff, stilted gait. Increased loss associated with the gastrointestinal or the urinary system is a common cause of hypokalemia, as indicated in Table 23.8. Differentiating gastrointestinal from urinary causes of hypokalemia is largely accomplished by clinical signs and physical exam, but fractional potassium excretion rates (FEK) may also be useful. Fractional potassium excretion can be calculated using the following formula: Table 23.8 Causes of hypokalemia. Source: Adapted from DiBartola, 1992a. Reproduced with permission of Elsevier. where U indicates the urine concentration of potassium (K+) or creatinine (CR), and S indicates the serum concentration. Treatment of hypokalemia is indicated if significant potassium loss is expected based on history and clinical signs (e.g., vomiting, diarrhea, overzealous use of diuretics) or if clinical signs of hypokalemia are present. Appropriate potassium administration is often required with prolonged fluid therapy. If feasible, oral potassium supplementation is most desirable since this is the safest route of administration. If intravenous potassium supplementation is warranted, the amount administered should be based on clinical status of the animal and measured serum potassium values. Table 23.9 provides approximate potassium dosages for treatment of hypokalemia in small animals. Alternatively, a rule of thumb may be applied in which 20 mEq/l of potassium is supplemented with careful monitoring of changes in serum potassium. An important caution regarding the administration of intravenous potassium is not to exceed a rate of 0.5 mEq/kg/h. Parenteral potassium administration should always be monitored to ensure that rate of potassium addition does not exceed rate of potassium movement into cells. Table 23.9 Potassium supplementation in treatment of hypokalemia aQuantity of potassium to add per liter of fluid. Do not exceed administration rate of 0.5 mEq K+/kg/h. Blood pH is highly regulated and is normally maintained between 7.38 and 7.42. Pulmonary and renal functions are necessary for precise regulation of pH of all body fluids, blood, and extravascular tissues. An acid is defined by Bronsted and Lowry as a substance that can supply H+ (protons), and a base is defined as a substance that can accept H+. In aqueous solutions, H+ are hydrated; therefore, H3O+ is considered an acid and is implied by the symbol H+. Blood pH is the negative logarithm of the hydrogen ion concentration. Although hydrogen ion concentration cannot be measured directly, hydrogen ion activity is measured chemically using a pH electrode. In body fluids, the difference between activity of hydrogen ions and concentration of hydrogen ions is negligible; hence hydrogen ion concentration and pH are commonly referred to in acid–base discussions. The hydrogen ion concentration of blood at pH 7.4 is 40 nmol/l (nanoequivalents per liter) and is therefore approximately a millionfold lower than the blood concentration of electrolytes such as sodium and potassium. Appropriate hydrogen ion concentration is critical in order to maintain body proteins in configurations required for enzymatic and structural function. An increase in hydrogen ion concentration with a decrease in blood pH is termed acidemia and can be caused by pathophysiological processes that cause accumulation of acids in the body. As the concentration of hydrogen ions decreases, and blood pH increases, alkalemia occurs and can be associated with pathophysiological processes that cause accumulation of alkali in the body. The disordered processes leading to acidemia and alkalemia are termed acidosis and alkalosis, respectively. On a daily basis, an excess of acid (70–100 mEq) is generated in the body as a result of dietary intake and intermediary metabolism. Catabolism of carbohydrate, fat, and protein account for most of this as a result of: oxidation of sulfur-containing amino acids to sulfuric acid; oxidation of phosphoproteins to phosphoric acid; incomplete oxidation of fats and carbohydrates to organic acid; production of lactate/lactic acid during anaerobic glycolysis; and conversion of carbon dioxide and water produced in the tricarboxylic acid cycle to carbonic acid. Buffers throughout the body minimize changes in blood pH associated with alterations of acid–base balance. The most effective physiological buffers have pKa values between 6.1 and 8.4, with buffering capacity being maximal within one pH unit of the pKa. Important extracellular buffers include bicarbonate, inorganic phosphates, and plasma proteins. Most extracellular buffering occurs as a result of the bicarbonate–carbonic acid buffer pair (pKa = 6.1). Equilibrium of this buffer pair is indicated with the following: The hydration of CO2 is a rapid reaction in the presence of the enzyme carbonic anhydrase, which is found primarily in red blood cells and renal tubular cells. The dissociation of any acid, in this case carbonic acid, can be described utilizing the concept that the velocity of a reaction is proportional to the product of the concentration of the reactants. In the case of the bicarbonate buffer system, the carbonic anhydrase–catalyzed hydration of CO2 to form H2CO3 reaches equilibrium almost instantaneously, with the number of dissolved CO2 molecules far exceeding the number of carbonic acid molecules. By defining dissociation constants and rearranging, the useful Henderson–Hasselbalch form of the dissociation equilibrium equation can be derived:
Principles of Acid–Base Balance: Fluid and Electrolyte Therapy, Blood Substitutes
Composition and Distribution of Body Fluids
Units of Measure
Term
Abbreviation
Description and conversion
Molecular (formula) weight
MW
Sum of atomic weights of all elements in a chemical formula
Millimole
mmol (mM)
Molecular (formula) weight of a substance in mg, equals 1 mM
Milliequivalent
mEq
Weight, in mg, of an element that combines or replaces 1 mg (1 mmol) of hydrogen (H+)
Milliosmole
mOsm
Always contains 6.0 × 1023 molecules and equals 1 mmol of a nondissociable substance
Milliequivalent per liter
mEq/l
Body Fluid Compartments
Compartment
% Body weight
Method
Total body water (TBW)
60
Indicator substance
Extracellular fluid (ECF)
20–27
Indicator substance
Red blood cells (RBC)
3
Counted + calculations
Plasma volume (PV)
5
Indicator substance
Total blood volume (BV)
5.7–10
Calculated: RBC volume + PV
Interstitial lymph fluid
15
Calculated: ECF − BV
Transcellular fluid
1–6
Estimated
Bone and dense connective tissue
5
Estimated
Intracellular fluid (ICF)
33–40
TBW − ECF
Species
Total blood volume
Plasma volume
RBC volume
Dogs
8.5
4.5
4.0
Cats
6.7
4.7
2.0
Chickens
6.5
4.5
2.0
Cattle
5.7
3.8
1.9
Goats
7.0
5.4
1.6
Horses
Draft
7.0
4.0
3.0
Thoroughbred
10.0
6.0
4.0
Saddle
7.7
5.2
2.5
Pigs
7.5
4.8
2.7
Sheep
6.5
4.5
2.0
Fluid and Electrolyte Distribution
Cations
mEq/l
Anions
mEq/l
Sodium
135–160
Chloride
110–125
Potassium
3–5
Bicarbonate
18–22
Calcium (total calcium 5–10 mM/l)
4–6
Phosphate
1–3a
Magnesium
1–3
Sulfate
1–2
Trace elements
1
Lactate
1–2
Other organic acids
3–5
Protein
10–16
Total
144–175
144–175
Water, Sodium, and Chloride
Homeostasis
Renal Regulation of Sodium, Chloride, and Water Excretion
Disorders of Water, Sodium, and Chloride Balance
Types of Dehydration
Percent dehydration
Clinical signs
4 or less
History of fluid loss, mucous membranes still moist, evidence of thirst
5–6
Subtle loss of skin elasticity, slight delay in return of skin to normal position, hair coat dull, mucous membranes slightly dry but tongue still moist
7–8
Definite delay in return of skin to normal position, both mucous membranes and tongue may be dry, eyeballs may be soft and sunken, slight prolongation of capillary refill time
9–11
Tented skin does not return to normal position, definite prolongation of capillary refill time, eyes definitely sunken in orbits, all mucous membranes dry, may be signs of shock such as tachycardia, cool extremities, rapid and weak pulses
12–15
Definite signs of shock and circulatory collapse, death is imminent
Hypernatremia
Hyponatremia
Hyperchloremia
Hypochloremia
Potassium
Homeostasis
Renal Regulation of Potassium Excretion
Disorders of Potassium Balance
Hyperkalemia
Decreased excretion
Urethral obstruction
Ruptured bladder
Anuric or oliguric renal failure
Hypoadrenocorticism
Gastrointestinal diseases (e.g., trichuriasis, salmonellosis, perforated duodenal ulcers)
Chylothorax with repeated drainage of the pleural effusion
Drugs
ACE inhibitors (e.g., captopril, enalapril)
Potassium-containing drugs (e.g., potassium chloride)
Potassium-sparing diuretics (e.g., spironolactone, amiloride,triamterene)
Nonsteroidal antiinflammatory agents
Heparin
Translocation from the intracellular to extracellular fluid
Acute mineral acidosis (e.g., HCl or NH4Cl administration)
Insulin deficiency (e.g., diabetic ketoacidosis)
Ischemia reperfusion
Drugs (e.g., propranolol)
Acute tumor lysis syndrome
Hyperkalemic periodic paralysis (rare)
Increased intake (rare)
Pseudohyperkalemia
Thrombocytosis
Hemolysis
Establish venous access and administer potassium-deficient fluids
Discontinue potassium intake, including drugs that may promote hyperkalemia
Administer the following as needed:
NaHCO3 (0.5–1 mEq/kg, slowly IV) if animal is acidotic
Calcium gluconate (10% solution; 0.5–1 ml/kg slowly IV up to10 ml maximum)
Glucose (20% solution; 0.5–1.0 g/kg IV)
Insulin (0.5 IU/kg) and glucose (20% solution; l g/kg; half givenIV bolus and the remainder infused over 2 hours)
Potassium-wasting diuretics (furosemide, chlorothiazide, hydrochlorothiazide)
Sodium polystyrene (20 g with 100 ml 20% sorbitol) per os or 50 g in 100–200 ml tap water (retention enema)
Peritoneal dialysis (last resort)
Hypokalemia
Increased loss
Gastrointestinal (FEK <4–6%)
Persistent vomiting of stomach contents
Diarrhea
Urinary (FEK >4–6%)
Chronic renal failure in cats
Diet-induced hypokalemic nephropathy in cats
Renal tubular acidosis
Postobstructive diuresis
Excess circulating mineralocorticoid
Hyperadrenocorticism
Primary hyperaldosteronism (hyperplastic or neoplastic)
Diuretics (loop acting, thiazides and osmotic)
Antibiotics (penicillins, amphotericin B, aminoglycosides)
Translocation from extracellular fluid to intracellular
Alkalemia
Overadministration of insulin and glucose-containing fluids
Hyperthyroidism
Hypokalemic periodic paralysis
Possible complication of hypothermia
Decreased intake
Unlikely as sole cause
Serum potassium concentration (mEq/l)
Supplement fluids (mEq/l)a
3.5 to 4.5
20
3.0 to 3.5
30
2.5 to 3.0
40
2.0 to 2.5
60
<2.0
80
Principles of Acid–Base Metabolism
Homeostasis
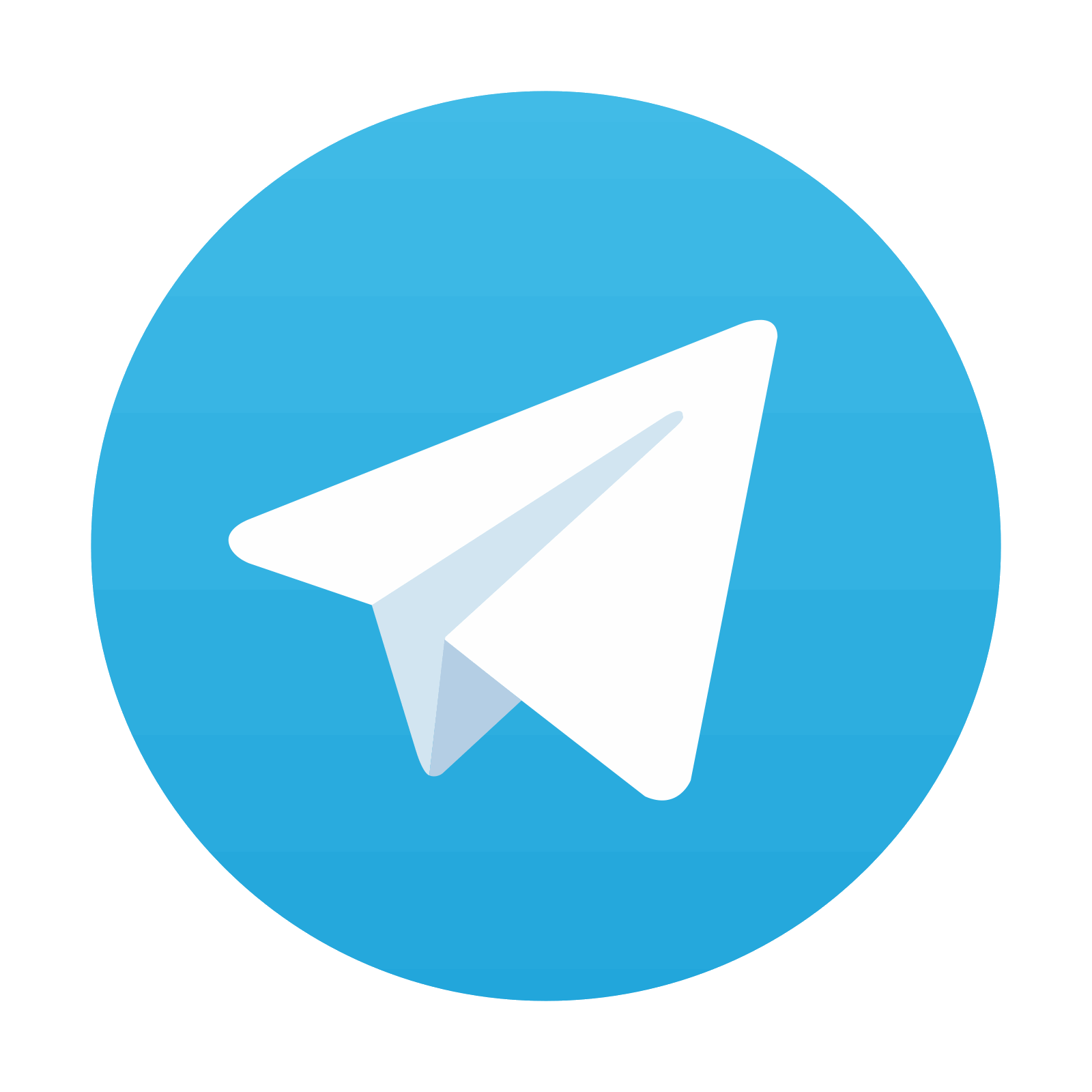
Stay updated, free articles. Join our Telegram channel
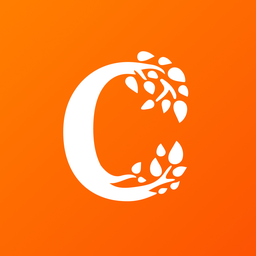
Full access? Get Clinical Tree
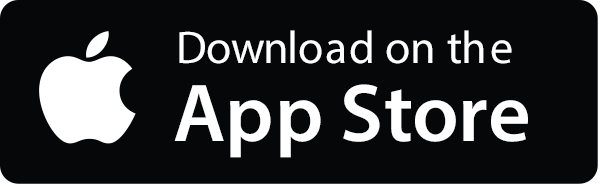
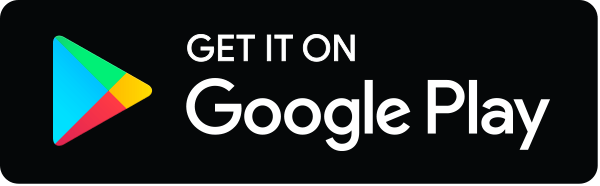