and Akihiko Takashima1
(1)
Lab. for Alzheimer’s Disease, RIKEN Brain Science Institute, Saitama, Japan
Abstract
Since the identification of the mutations in presenilin 1 and presenilin 2 genes more than a decade ago, a great deal of research has filled the gap in our knowledge of mutations underlying various phenotypes of Alzheimer’s disease (AD) that appear relatively early in the life of presenilin (PS) mutation carriers. Various molecular and cell biological techniques that showed functional differences between wild-type and mutant PS emerged during this time. In this chapter, we review this research by roughly categorizing findings that are similar or support a certain hypothesis. Sect. 1 discusses the function of PS as the component of γ-secretase, which generates amyloid β (Aβ). We also present a short history of how PS mutations were first considered to produce more Aβ42 (gain of function) and later found to produce less Aβ40, resulting in a higher Aβ42/Aβ40 ratio (loss as gain of function). This produces a condition in which Aβ is prone to aggregate, supporting the amyloid hypothesis of AD. Sect. 2 summarizes PS function vis-à-vis Notch signaling, which was identified by the gene-knockout approach. A hypothesis is discussed that suggests a partial loss of function, mainly based on various AD-like phenotypes observed in conditional double PS knockout mice. The first half of Sect. 3 is devoted to a review of various abnormalities related to the intracellular calcium regulation in cell and animal transgenic models of PS. The remainder of Sect. 3 discusses other potential mechanisms of PS dysfunction caused by mutations. These include abnormalities in protein trafficking, β-catenin/cadherin-related activities, and posttranslational modifications, the latter of which include endoproteolytic cleavage of PS itself, GSK-3β-dependent phosphorylation of tau, autophagy-based protein degradation, neprilysin-mediated Aβ metabolism, and alteration of unfolded protein response signaling. Any one or more of these PS dysfunctions may underlie the pathogenesis of familial AD and perhaps sporadic AD, if linked to mode of actions caused by nongenetic risk factors such as aging. Finally, we suggest the importance of bridging nonlinear dynamics of memory with molecular neuroscience of AD from multidimensional perspectives.
Key words
PresenilinAβAPPTauGain of functionLoss as gain of functionLoss of functionFamilial Alzheimer’s diseaseNotch signalingTraffickingGSK-3βAutophagyβ-cateninN-cadherinNeprilysinUnfolded protein responseTransgenicKnockoutKnock-inAgingγ-secretaseCalciumExcitotoxicityAmyloid hypothesisPresenilin hypothesisCalcium hypothesisAmyloid oligomer1 The Amyloid Hypothesis: Role of Familial Alzheimer’s Disease-Linked Presenilin Mutations
1.1 Formulation of the Amyloid Cascade Hypothesis
The amyloid hypothesis is the most popular hypothesis concerning the pathogenesis of Alzheimer’s disease (AD). This hypothesis states that the accumulation of longer form(s) of amyloid β (Aβ) in the brain is the primary influence driving AD pathogenesis (1, 2). One of the known physiological functions of presenilin (PS) is to cleave Aβ from its precursor protein (i.e., amyloid precursor protein, APP). We summarize first how the amyloid hypothesis was formulated within this context.
The first missense mutation associated with familial cases of AD (FAD) was found in the APP gene (3). Located on the long arm of chromosome 21, this mutation causes an amino-acid substitution from Val to Ile close to the carboxy terminus of Aβ. A few years later, Aβ was isolated from conditioned medium of human neuroblastoma cells transfected with constructs expressing wild-type APP or APP717 mutants linked to FAD (4). Analysis of Aβ by ELISA demonstrated that the APP717 mutations consistently caused a 1.5- to 1.9-fold increase in the percentage of longer Aβ species generated. The hydrophobic nature of a few residues at the carboxy terminus of Aβ is thought to make longer Aβ species, such as Aβ42, to be more prone to aggregate than shorter species like Aβ40, which constitutes the major species of the heterogeneous species generated (5, 6).
Increased levels of longer Aβ species generated from mutant APP indicated that FAD-linked APP mutants may cause AD by fostering amyloid deposition and the formation of senile plaques, a pathological hallmark believed to be directly correlated with disease symptoms (4). Immunostaining with carboxy terminus-specific antibodies against both Aβ40 and Aβ42(43) revealed that diffuse plaques, representing the earliest stage of Aβ deposition in the cortex of AD patients, exclusively stained positive for Aβ42(43) but negative for Aβ40 (7). This shows that one of the first pathological Aβ species deposited is Aβ42(43), the longer form (7). To test the absolute requirement of Aβ42 for amyloid deposition, transgenic models were generated that express Aβ40 or Aβ42 in the absence of human APP overexpression (8). While mice expressing high levels of Aβ40 did not develop overt amyloid pathology, mice that express a 5- to 10-fold lower Aβ42 transgene compared to mice expressing Aβ40 accumulated insoluble Aβ42 and developed both diffuse and compact amyloid plaques, confirming that Aβ42 is an essential component of amyloid deposition in mice. According to the modified version of the amyloid hypothesis, soluble amyloid oligomers, not amyloid deposits, are toxic and may contribute to AD pathogenesis (9, 10).
1.2 From “Gain of Function” to “Loss as Gain of Function”
A year after the identification of a FAD-linked mutation in the APP gene, genetic linkage analysis pointed to a FAD locus on chromosome 14 (11). Three years later, a PS1 gene bearing five different missense mutations was found to cosegregate with early-onset FAD (12). Several point mutations in a similar gene (i.e., PS2) were soon identified on chromosome 1 (13–15). On the basis of these results, it was established that mutations in the genes encoding APP, PS1, and PS2 cause early-onset, autosomal dominant AD. To establish that PS mutations act as gain-of-function dominants, researchers constructed mice expressing wild-type and mutant PS genes (16). Overexpression of mutant, but not wild-type PS1, selectively increases brain Aβ42(43), suggesting that PS mutations probably cause FAD through a gain of function that increases the amount of Aβ42(43) in the brain (16). This assumption was supported by a report of elevated Aβ42(43) in plasma from subjects with FAD-linked PS1, PS2, and APP mutations (17).
Another group established transfected cell and transgenic mouse models that coexpress human PS and APP genes and analyzed quantitatively the effects of PS expression on APP processing (18). In the transfected cells, PS1 and PS2 mutations caused a highly significant increase in Aβ42 secretion in all mutant clones. Likewise, mutant but not wild-type PS1 transgenic mice showed significant overproduction of Aβ42 in the brain. It was also demonstrated that transgenic animals that express both mutant PS1 (A246E) and Swedish mutant APP (APPswe) develop numerous amyloid deposits much earlier than age-matched control mice expressing APPswe and wild-type human PS1 or APPswe alone (19). These results provided evidence for the view that FAD-linked mutant PS1 causes AD through a pathogenic mechanism that accelerates the rate of Aβ deposition in brain. Consistent with this idea is the finding of accelerated plaque accumulation and associative learning deficits in mice carrying both the PS1 A246E mutation and APP K670N/M671L (20).
In another series of transgenic mice that coexpress APPswe with two FAD-PS1 variants that differentially accelerate amyloid pathology in the brain, a direct correlation was found between the concentration of Aβ42 and the rate of amyloid deposition (21). They also showed that the shift in Aβ42/Aβ40 ratio associated with the expression of FAD-PS1 variants is due to a specific elevation in steady-state levels of Aβ42, while maintaining a constant level of Aβ40 (21). Other evidence supporting the idea that clinically manifested PS1 mutations cause a gain of function is found in studies investigating whether PS1 is involved in normal APP processing. In neuronal cultures derived from PS1-deficient mouse embryos, cleavage of the extracellular domain of APP by α- and β-secretase was not affected by the absence of PS1, whereas cleavage of the transmembrane domain of APP by γ-secretase was partially prevented (22). This incomplete cleavage caused carboxy-terminal fragments (CTF) of APP to accumulate and a fivefold drop in the production of Aβ peptides. One caveat was the important residual γ-secretase activity observed, which led to the question: Do cells that are completely devoid of PS1 and PS2 maintain this γ-secretase activity? This question was subsequently addressed using stem cells.
Because PS-null mice die early in embryogenesis, pluripotent lines of embryonic stem cells from PS-null blastocysts were generated by mating of PS1+/– × PS2–/– mice (23, 24). After confirming the absence of PS1 and PS2 by Western blotting, Herreman et al. expressed APP695 harboring the K595N/M596L (Swedish) mutation in embryonic stem cells using recombinant Semliki Forest virus. In contrast to results obtained with single-knockout cells, no Aβ was detected in the conditioned media of PS1–/–PS2–/– cells. Similar observations were reported by comparing Aβ production from PS1+/+PS2+/+, PS1–/–PS2+/+, PS1+/–PS2–/–, and PS1–/–PS2–/– blastocyst cultures (25).
The reports described are just part of the evidence that more or less supports the view that increased production of Aβ42 due to mutations in PS genes contributes to AD pathogenesis. This view, which is often referred to as “gain of function” as a result of PS mutations, is consistent with the consequences of APP mutations and therefore strongly supports the amyloid hypothesis.
Contemporaneously, other reports appeared that were less well recognized but nonetheless described the phenotypes of FAD mutations from another perspective, one that focused on the ratio of Aβ species. One study using cell models showed that the FAD-linked APP mutation V715M significantly reduces Aβ40 secretion without strongly affecting Aβ42 production (26). The significance of an enhanced Aβ42/total Aβ ratio in cells expressing PS1 mutants compared to that in wild-type cells was also recognized (27). In vitro aggregation kinetics of a mixture of Aβ40 and Aβ42 also lends support to this view. With a specific ratio in which Aβ42 is increased and Aβ40 is decreased, not only did we observe accelerated nucleation, but we also observed induced elongation and cytotoxicity (28). Using PS1/PS2 mouse embryonic fibroblast cells and transient transfection with PS constructs, Schroeter et al. suggested that some FAD-causing PS mutations reside in proteins possessing low catalytic activity (29). Similarly, most PS2 mutations cause a significant decrease in Aβ40 and increase in the Aβ42/Aβ40 ratio (30). Another comprehensive analysis found that mutations such as Dexon9 and L166P in PS1 cause a reduction in Aβ40 production, whereas G384 mutant significantly increases Aβ42, suggesting that the different mutations in PS affect γ-secretase structure or function in different ways (31). In HEK293 cells stably expressing PS with or without various mutations, all mutations significantly increased the Aβ42/Aβ40 ratio by decreasing Aβ40 and causing the accumulation of APP CTF fragments, a sign of decreased PS activity (32). In this system, a significant increase in the absolute levels of Aβ42 was observed for only half of the mutations tested. Moreover, this study also showed that the age of onset of PS1-linked FAD correlated inversely with Aβ42/Aβ40 and absolute levels of Aβ42, but directly with Aβ40 levels. Cell lines from PS1/PS2-deficient fibroblasts stably transfected with either wild-type PS1 or different mutants also showed that the total amount of Aβ secreted by FAD mutant PS1-expressing cells is significantly reduced, whereas the Aβ42/Aβ40 ratio is increased relative to wild-type PS1-expressing cells (33).
These consistent in vitro observations on the consequences of PS1 mutations on Aβ42/Aβ40 ratios were further corroborated with mouse models. PS1 knock-in mice, in which exon 10, which encodes most of the hydrophilic loop sequence, was artificially deleted from the endogenous PS1 gene, were reported to be viable but exhibited drastically reduced γ-secretase cleavage at the Aβ40 site but not at the Aβ42 site (34). Surprisingly, this reduction of Aβ40 was associated with exacerbated plaque pathology when expressed on an APP transgenic background, suggesting that decreased Aβ40, not increased Aβ42, is likely to be the cause of accelerated plaque deposition in these animals. The same group also expressed the PS1 FAD-linked mutant M146V knock-in allele, either on wild-type PS1 or PS1 null backgrounds, and crossed these mice with Tg2576 APP transgenic mice (35). Introducing the PS1 M146V mutation on a Tg2576 background caused an earlier onset of plaque pathology, whereas removing wild-type PS1 in the presence of the PS1 M146V mutation greatly exacerbated the amyloid burden. This was attributed to a reduction of γ-secretase activity rather than an increase in Aβ42. In another study, BRI-Aβ40 mice that selectively express a fusion protein between the BRI protein, involved in amyloid deposition in Familial British (FBD) and Danish Dementia (FDD) and Aβ40 were crossed with both Tg2576 mice and BRI-Aβ42 mice expressing a fusion protein between the BRI protein and Aβ42 (8, 36). In the bitransgenic mice, the increased steady-state levels of Aβ40 decreased Aβ deposition by 60–90%, demonstrating that Aβ42 and Aβ40 have opposing effects on amyloid deposition: Aβ42 promotes amyloid deposition, whereas Aβ40 inhibits it. Furthermore, because increasing Aβ40 levels protected BRI-Aβ40/Tg2576 mice from the premature-death phenotype observed in Tg2576 mice, the authors suggested that strategies that preferentially target Aβ40 might actually worsen the disease course and that a selective increase in Aβ40 levels may actually reduce the risk for development of AD (36). However, since increasing Aβ40 levels (BRI-Aβ40/BRI-Aβ42 mice) robustly exacerbated the premature-death phenotype in BRI-Aβ42 mice, their assumption may not simply apply in terms of mortality.
Regardless, these studies importantly observed that PS mutation-linked FAD is not always caused by increased Aβ42 (i.e., gain of function), but instead is more often caused by reduced Aβ40, resulting in an increased Aβ42/Aβ40 ratio (i.e., loss as gain of function). Although consensus has not been reached at this point, there is certainly a trend toward considering PS mutations in AD pathogenesis as more than a simple gain-of-function assumption to a loss-as-a-gain-of-function (37–39).
2 The Presenilin Hypothesis of Alzheimer’s Disease
2.1 Understanding the Physiological Function of Presenilin
A possible counterpart of the original gain-of-function hypothesis is the “loss-of-function” hypothesis, which is based on the idea that FAD-linked mutations cause PS dysfunction (38). There is a short history of investigations that led to the formation of this hypothesis, which occurred independent of the amyloid hypothesis. We imagine that the loss-of-function hypothesis was primarily formulated on the basis of the plausible logic that understanding the physiological function of PS should reveal the underlying mechanisms of AD. Indeed, FAD is caused by the dysfunction of PS as a result of gene mutation. One way to understand the normal biological function of a protein is by knocking out the gene responsible for producing the protein in organisms (40). A targeted null mutation in the murine homolog of PS1 (PS1–/– mice) causes mice to die shortly after birth (41). Homozygous PS1–/– mice have deformed skeletons, extensive CNS hemorrhages, thin ventricular zone, and bilateral cerebral cavitations, suggesting that PS1 is required for proper formation of the axial skeleton, normal neurogenesis, and neuronal survival (41).
PS1 is known to be a functional homologue of Caenorhabditis elegans sel-12, which facilitates signaling mediated by the Notch/LIN-12 family of receptors (42). Another group generated PS1–/– mice with the goal of examining potential roles of PS1 in facilitating Notch-mediated signaling during mammalian embryogenesis (42). PS1–/– embryos exhibited abnormal patterning of the axial skeleton and spinal ganglia as well as reduced expression of mRNA encoding Notch1 and Delta-like 1 (Dll1), a vertebrate Notch ligand in the presomitic mesoderm (42). This suggested that PS1 is required for the spatiotemporal expression of Notch1 and Dll1, which are essential for somite segmentation and maintenance of somite borders (42). Another group reported that PS1–/– mice develop a cortical dysplasia resembling human type 2 lissencephaly, with leptomeningeal fibrosis and migration of cortical-plate neurons beyond their normal position into the marginal zone and subarachnoid space (43). This disorder of neuronal migration is associated with the disappearance of the Cajal-Retzius pioneer neurons accompanied by disorganization of Notch1 immunoreactivity on the neuronal cell membranes (43). Because PS1 is normally expressed in the leptomeninges, the authors suggested that the loss of Cajal-Retzius cells was caused by a defective trophic interaction with leptomeningeal cells, possibly involving disruption of Notch signaling. Another study also showed that PS1-mediated Notch-signaling is required to induce Dll1 expression in the caudal half of the somite, indicating that PS1-dependent activation of the Notch-signaling pathway is necessary to regulate Dll1 expression and to establish the rostrocaudal polarity of somites (44). On the other hand, in the ventricular zone of the PS1–/– mice generated by Shen et al. (41), expression of the Notch1 downstream effector gene Hes5 is reduced and expression of the Notch1 ligand Dll1 is elevated, whereas expression of Notch1 is unchanged (45). The level of Dll1 transcripts is also increased in the presomitic mesoderm of PS1–/– embryos, while the level of Notch1 transcripts is unchanged (45). Since their mice also showed premature differentiation of neural progenitor cells, the authors suggested that PS1 controls neuronal differentiation in association with the downregulation of Notch signaling during neurogenesis (45). Abnormal blood vessel development in PS1–/– mouse embryos has also been reported, suggesting the essential roles of PS1 in angiogenesis as well (46).
Mice homozygous for a targeted null mutation in PS2 (PS2–/– mice) exhibit no obvious defects, whereas loss of PS2 on a PS1 null background (PS1–/–PS2–/– mice) leads to early embryonic lethality and multiple early patterning defects, including lack of somite segmentation, disorganization of the trunk ventral neural tube, midbrain mesenchyme cell loss, anterior neuropore closure delays, and abnormal heart and second brachial arch development (47). Additionally, in PS1–/–PS2–/– mice, Hes5 expression is undetectable and Dll1 is expressed ectopically in the neural tube and brain of embryos, supporting the premise that PS plays essential roles in Notch signaling (47). Another report showed that PS2–/– mice develop only mild pulmonary fibrosis and hemorrhage with age (24). Although PS1+/–PS2–/– mice survive with relatively good health, PS1–/–PS2–/– mice display a phenotype closely resembling full Notch1 deficiency, suggesting that PS1 is essential and PS2 is redundant for normal Notch signaling during mammalian embryological development (24). PS1+/–PS2–/– mice also develop splenomegaly with severe granulocyte infiltration, suggesting a critical role of PS in myelopoiesis (48). Six months after birth, the majority of PS1+/–PS2–/– mice develop an autoimmune disease characterized by dermatitis, glomerulonephritis, keratitis, vasculitis, hypergammaglobulinemia, and benign skin hyperplasia, indicating that skin and immune systems are especially sensitive targets of impaired PS function (49).
There are other mouse models in which the Thy-1 promoter was used to drive PS1 expression in order to rescue the embryonic lethality of PS1 knockout mice, resulting in organ-specific knockouts (50, 51). In a skin-specific deficient model, loss of PS1 caused epidermal dysplasia and skin cancer, which was suggested to result from β-catenin accumulation (50). In a kidney-specific deficient model, lack of both PS1 and PS2 resulted in altered nephrogenesis, most likely because of deficient Notch signaling (51). Overall, it is clear that genetic deficiencies in PS affect a variety of physiological functions, mainly via the Notch signaling pathway, but other pathways might contribute as well (40).
2.2 Partial Loss of Presenilin Function as a Mechanistic Model of Alzheimer’s Disease
Having seen a great deal of evidence suggesting that genetic deficiencies in PS cause a variety of developmental abnormalities, mainly via Notch signaling, one might question how these abnormalities may or may not relate to the pathogenesis of AD. An attempt to answer this question was made by overcoming the embryonic lethal characteristics of PS knockout mice again genetically. Selective inactivation of PS1 in the forebrain has been achieved by crossing floxed PS1 gene mice (PS1cKO) with mice expressing Cre recombinase under the control of the αCaMKII promoter (52, 53). PS1cKO mice show no gross abnormalities but do accumulate the CTF of APP and display reduced generation of Aβ (52). The expression of Notch downstream effecter genes, Hes1, Hes5, and Dll1, is unaffected in the cerebral cortex of these mice (52). Although basal synaptic transmission and synaptic plasticity, a hypothetical cellular coordinate of memory, such as long-term potentiation (LTP) and long-term depression (LTD) at CA1 synapses are normal, PS1cKO mice exhibit subtle but significant deficits in long-term spatial memory (53). Another report showed that PS1cKO mice have a pronounced deficiency in enrichment-induced neurogenesis in the dentate gyrus, even though this reduction in neurogenesis does not result in LTP or learning deficits, indicating that the addition of new neurons is not required for memory formation (53). However, the higher extent of memory retention in PS1cKO mice than in control mice after postlearning enrichment suggests that adult dentate neurogenesis may play a role in the periodic clearance of outdated hippocampal memory traces after cortical memory consolidation, thereby ensuring that the hippocampus is continuously available to process new memories (53). Because PS1cKO mice still possess γ-secretase activity of PS2, the possible contribution of Aβ to their memory deficits cannot be excluded. Thus, forebrain-specific PS1cKO mice were generated on a PS2–/– background (PS1cKO PS2–/– or PScDKO) (54). PScDKO mice lacking both PS1 and PS2 in the postnatal forebrain exhibit impaired hippocampal memory and synaptic plasticity linked to specific reductions in NMDA receptor-mediated responses (54). They also show reduced synaptic levels of NMDA receptors, αCAMKII, cAMP response element binding protein (CREB) binding protein (CBP), as well as CREB/CBP target gene products such as c-fos and brain-derived neurotrophic factor (BDNF) (54). With increasing age, these mutant mice develop striking neurodegeneration of the cerebral cortex and worsening impairments of memory and synaptic function (54). Neurodegeneration is accompanied by increased levels of p25 that activates cyclin-dependent kinase 5 (Cdk5), one of the kinases phosphorylating tau, and hyperphosphorylated tau (54). The authors speculated that PS is needed for the correct transport and insertion of the affected NMDA receptors into the postsynaptic membrane (54).
Decreased NMDA receptor-signaling could explain the decreased αCaMKII activity and the changes in LTP and synaptic transmission observed in PScDKO mice. Abnormal Notch signaling could also contribute to the overall phenotype of these mice. CBP contains a consensus sequence site for the CBF-1 transcription factor, which is regulated by Notch (54). CBP is an essential cofactor for the transcription factor CREB, and deficiencies of these factors on their own lead to neurodegeneration (54). Although these speculations (54) still have to be confirmed, especially in the context of age-dependency, the various phenotypes of PScDKO mice that are also seen in AD patients (with the exception of Aβ deposition), as well as the observation that PS mutations do not increase Aβ42 but decrease Aβ40, have provided the main rationale for proposing that partial loss of PS function underlies memory impairment and neurodegeneration in the pathogenesis of AD (55).
The molecular events that may underlie the observed phenotypes were further investigated (56). ELISA analysis showed that both PS1cKO and PScDKO mice had reduced levels of Aβ40 and Aβ42, although any change in the ratio of the two Aβ species was not mentioned. Microarray, quantitative real-time reverse transcription PCR, and Western blot analyses confirmed the elevated levels of glial fibrillary acidic protein, complement component C1q, and cathepsin S (56), the upregulation of which has been associated with inflammatory responses in various neurodegenerative processes (56). Strong microglial activation was also observed in the hippocampus and deep cortical layers of PScDKO mice (56). These results support the premise that the memory impairment and neurodegeneration in PScDKO mice are not caused by Aβ accumulation and that loss of PS function leads to differential upregulation of inflammatory markers in the cerebral cortex.
In C. elegans lacking sel-12, six types of FAD-linked mutant PS1 had a reduced ability to rescue the phenotype, suggesting that mutant PS1 has lower than normal PS activity (57). A similar study also showed that human wild-type PS1, not mutant PS1, fully rescued the sel-12-deficient phenotype, namely an egg-laying defect, supporting the hypothesis that PS1 mutations cause a partial loss of PS1 function (58). Mutations of sel-12 in C. elegans result in a defect in temperature memory that is caused by the loss of PS function in two cholinergic interneurons that display neurite morphology defects (59). The morphology of the affected neurons in sel-12 mutant animals can be rescued by expressing only wild-type human PS1, not FAD mutant PS1 A246E, in these cells (59). In contrast, in PS1–/– mice, embryonic lethality and axial skeletal defects were efficiently rescued by both human wild-type PS1 and A246E PS1 to similar degrees, findings consistent with the view that FAD-linked PS1 mutants retain sufficient normal function during mammalian embryonic development (60, 61). Furthermore, a 50% reduction of PS1 activity in PS1+/– mice does not lead to Aβ42(43) increase, whereas expression of human mutant PS1 on a murine PS1 null background is sufficient to elevate Aβ42(43), supporting the idea that gain-of-function activity results from the PS1 mutation (61).
Although these pioneering reports in mice described much about the developmental abnormalities of these mice and about Aβ deposition, they did not rigorously examine the cognitive functions of these mice. In fact, the lack of progressive spatial learning impairment in mice expressing the mutated human PS1 (M146L and L286V) transgene in the Morris water maze test indicates that other AD-associated genes like APP may be required for full phenotypic expression of mutant PS1 alleles (62). At 3 months of age, PS1 M146V knock-in mice exhibited impaired hippocampus-dependent associative learning as measured with a contextual fear conditioning paradigm; this was correlated with reduced adult neurogenesis in the dentate gyrus, indicating that impaired adult neurogenesis may contribute to the memory deficit associated with FAD (63). Mice with postnatal neuron-specific PS1 deficiency (PS1 n–/–) were generated by means of loxP/Cre recombinase-mediated deletion (64). In adult PS1 n–/–mice, levels of endogenous brain Aβ are greatly reduced, concomitant with accumulation of APP CTF (64). Crossing APP V717I transgenic mice with PS1 n–/– mice effectively prevented the amyloid pathology and LTP deficit in APP V717I transgenic mice (64). However, the cognitive defect, assessed by the object recognition test, in APP single transgenic mice could not be rescued by crossing these mice with PS1 n–/– mice. Similarly, conditional inactivation of PS1 in APP transgenic mice (PS1cKO;APP Tg) also effectively prevents the accumulation of Aβ and inflammatory responses, although it also causes an age-dependent accumulation of APP CTF (65). Short-term PS1 inactivation in young PS1cKO; APP Tg mice rescued deficits in contextual fear conditioning and serial spatial reversal learning in a water maze (65). However, longer-term PS1 inactivation in older PS1cKO;APP Tg mice failed to rescue contextual memory and hippocampal LTP, and had a decreasing ameliorative effect on spatial memory impairment. Taken together, these observations highlight the complex functional relationship of mutant PS and APP to synaptic plasticity and memory in the adult and aging brain (64). Because of the complex nature of memory in the course of AD progression, it makes it difficult to determine which genetically manipulated animal is a better model for AD or FAD.
3 Various Forms of Presenilin Malfunction in Alzheimer’s Disease Pathogenesis
3.1 Calcium Dysregulation Hypothesis of Presenilin Mutations
Most reports that support the “gain-of-function” or “loss-as-a-gain-of-function” hypotheses are based on the idea that amyloid deposition results from an increased Aβ42/Aβ40 ratio. The recent discovery of a soluble oligomer as the most toxic species of Aβ requires these hypotheses to be adjusted so that they address whether PS mutations indeed promote the generation of Aβ oligomers and in what ways these mutations cause memory deficits. In addition, it still needs to be established how the lack of, or reduction in, normal physiological PS function (i.e., loss-of-function) mediated by Notch signaling and by other potential molecules cause AD-like phenotypes in aging animals. We will discuss these issues more in detail later in this section.
One shared view of both hypotheses is the relatively simplistic view that the mechanisms underlying PS mutation-related FAD may also apply to sporadic AD. If this were the case, then the increase in Aβ42/Aβ40 ratio and disruption of Notch signaling or other PS function in sporadic AD patients should be demonstrated before expanding the argument beyond genetics. The highest risk factor for AD is advanced age, not genetic dysfunction.
An AD pathogenesis hypothesis that takes into account both genetic and aging risk factors is the calcium dysregulation hypothesis (66). During aging, and particularly in neurodegenerative disorders, cellular calcium-regulating systems are compromised, resulting in synaptic dysfunction, impaired plasticity, and neurodegeneration. From the perspective of cell and animal models, we briefly summarize here only the genetic aspect, specifically those relating to PS mutations, of perturbed calcium homeostasis.
To explore a possible link between a genetic defect that causes AD and excitotoxic neuronal degeneration, Guo et al. generated PS1 mutant knock-in mice (PS1 M146V KI) (67). Although these mice displayed no overt mutant phenotype, their hippocampus was hypersensitive to seizure-induced synaptic degeneration and necrotic neuronal death. Moreover, cultured hippocampal neurons from PS1 M146V KI mice had increased vulnerability to glutamate-induced death, which was correlated with perturbed calcium homeostatis, increased oxidative stress, and mitochondrial dysfunction. Agents that suppress calcium influx/release and antioxidants protected neurons against excitotoxic action of the PS mutation. The same group showed that synaptosomes prepared from transgenic PS1 mutant mice exhibited elevated cytoplasmic calcium levels following exposure to depolarizing agents and Aβ (68). Mitochondrial dysfunction and caspase activation following exposures to Aβ were exacerbated in synaptosomes from PS1 mutant mice. Agents that buffer cytoplasmic calcium or prevent calcium release from the endoplasmic reticulum (ER) protected synaptosomes against the adverse effect of PS1 mutations on mitochondrial function.
In an electrophysiological study using hippocampal slices, medium and late afterhyperpolarizations in CA3 pyramidal cells were larger in mice overexpressing PS1 M146L or M146V than in wild-type and nontransgenic control mice (69). Calcium responses to depolarization and synaptic potentiation of the CA3-to-CA1 projection were also stronger in M146L mice than in nontransgenic littermates, demonstrating disruption of the control of intracellular calcium and electrophysiological dysfunction in PS1 mutant mice. Primary neurons from another line of PS1 mutant transgenic mice (L286V) also showed increased vulnerability to both excitotoxic and hypoxic-hypoglycemic damage compared to neurons from wild-type PS1 transgenic mice or nontransgenic mice (70). In addition, less excitotoxic damage occurred in neurons from PS1 knockout mice and wild-type mice in which PS1 gene expression was knocked down by antisense treatment (70).
Accelerated neuronal death was demonstrated in the hippocampus of mutant PS1 mice after peripheral administration of kainic acid in comparison to nontransgenic mice (71). Moreover, another line of human mutant PS1 transgenic mice (A246E), not human wild-type PS1 mice, showed (1) lower excitotoxic threshold for kainic acid in vivo, (2) greater hippocampal LTP in brain slices, and (3) increased glutamate-induced intracellular calcium levels in isolated neurons (71). Prominent higher calcium responses were triggered by thapsigargin and bradykinin, indicating that mutant PS modulates the dynamic release and storage of calcium ions in the ER. In reaction to glutamate, overfilled calcium stores resulted in higher than normal cytosolic calcium levels, explaining the facilitated LTP and enhanced excitotoxicity. Lower excitotoxic threshold for kainic acid was also observed in transgenic mutant human PS2 (N141I) mice; this lower threshold was prevented by dantrolene, an inhibitor of calcium release from the ER (71).
Fibroblasts obtained from mutant PS1 (M146V) knock-in mice showed a marked potentiation in the amplitude of calcium transients evoked by bradykinin, a cell surface receptor agonist (72). These cells also showed significant impairments in capacitative calcium entry (CCE), an important cellular signaling pathway wherein depletion of intracellular calcium stores triggers an influx of extracellular calcium into the cytosol. Notably, deficits in CCE were evident after bradykinin stimulation, but not if intracellular calcium stores were completely depleted with thapsigargin (72). Treatment with ionomycin and thapsigargin revealed that calcium levels within the ER were significantly increased in mutant PS knock-in cells (72). Collectively, these observations suggest that the overfilling of calcium stores represents the fundamental cellular defect underlying alterations in calcium signaling conferred by PS mutations (72). Another group followed up this study using stable PS1 SY5Y cell lines (73). PS1 knockout and inactive mutant PS1 D257A potentiated CCE, whereas mutant PS1 M146L and mutant PS2 N141I (primary embryonic cortical neurons derived from transgenic mice) significantly reduced CCE compared to wild-type PS. While inhibition of CCE by its antagonist SFK96365 selectively increased Aβ42, increased accumulation of Aβ had no effect on CCE. Thus, reduced CCE is likely to be an early cellular event leading to increased Aβ42 generation associated with FAD mutant PS (73).
Electrophysiological recordings with two-photon imaging revealed that mutant PS knock-in (PS KI M146V) mice displayed exaggerated ER calcium signals relative to nontransgenic mice (74). In nontransgenic mice, ryanodine receptors (RYRs) contributed modestly to inositol triphosphate (IP3)-evoked calcium release, whereas the exaggerated signals in PS1 KI (M146V) mice resulted primarily from enhanced RYR-mediated calcium release and were associated with increased RYR expression across all ages. The consequences of this abnormality in PS1 KI (M146V) mice include increased vulnerability of neurons to excitotoxic, metabolic, and oxidative insults (66).
< div class='tao-gold-member'>
Only gold members can continue reading. Log In or Register a > to continue
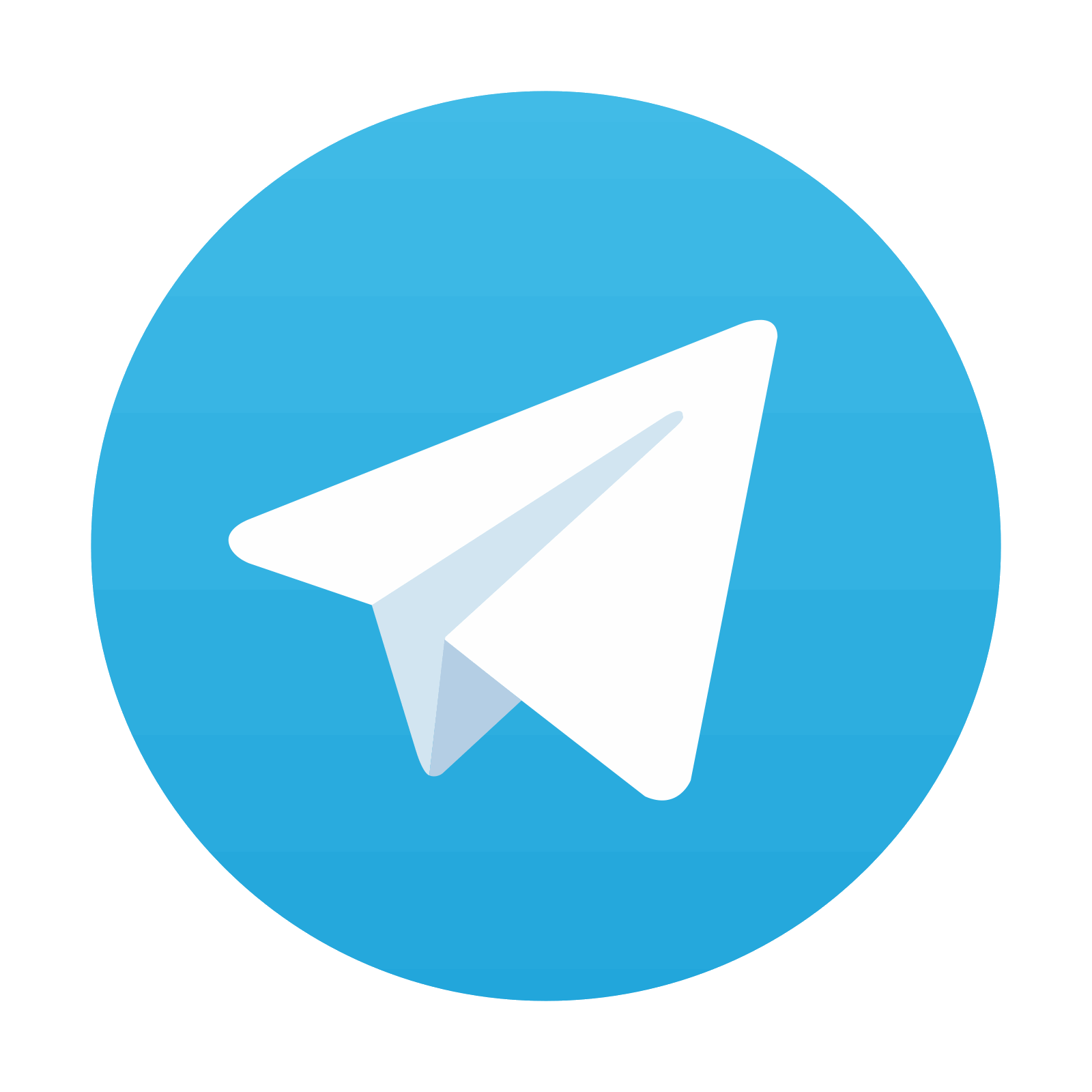
Stay updated, free articles. Join our Telegram channel
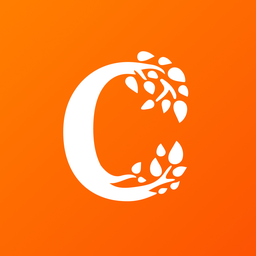
Full access? Get Clinical Tree
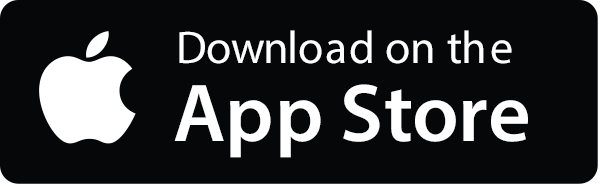
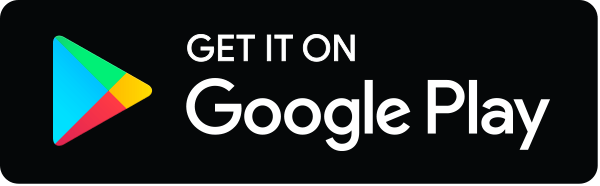