Chapter 7 Pharmacology of drugs used to treat cardiac disease
DRUGS USED IN THE TREATMENT OF MYOCARDIAL FAILURE
In compensated heart failure due to ineffective myocardial contractility, adaptive mechanisms of the cardiovascular system occur to sustain adequate blood pressure and cardiac output brought about by the sympathetic nervous system, the renin–angiotensin–aldosterone system and by other mediators (see Chapters 2 and 5) that bring about vasoconstriction as well as increases in circulating volume (increased preload), cardiac contractility, cardiac output and heart rate in order to maintain peripheral perfusion. In decompensated heart failure, due to ineffective myocardial contractility, there is little or no further augmentation of stroke volume with increasing filling pressures. Retrograde transmission of increased pressures into the systemic and pulmonic vasculature produces oedema. Vasoconstriction increases afterload, which further decreases cardiac output. Tachycardia from increased sympathetic drive decreases diastolic coronary blood flow. Myocardial hypoxia and excessive ventricular wall stress as a result of increased filling pressure contributes to the decline in stroke volume. The inotropic and chronotropic responses to sympathetic adrenergic stimulation and the increase in work required to pump against the elevated afterload increase myocardial energy expenditure and accelerate the rate of cell death. Oedema of the myocardial tissues produced by fluid retention contributes to poor myocardial function and impairs oxygenation. Oxygenation is also impaired by pulmonary oedema.
The goals in the treatment of heart failure are to decrease fluid retention, decrease afterload, improve contractility of the myocardium and decrease the heart rate. Three main classes of drugs are utilized: (1) vasodilators, (2) diuretics and (3) cardiac glycosides. In addition, iontropic drugs and pressor agents have a role in supporting ventricular function but these drugs are primarily used in supporting cardiac function in patients with circulatory shock or under anaesthesia (see Chapters 20 and 21).
Vasodilators
As cardiac output falls due to ineffective myocardial contractility, activation of the renin–angiotensin–aldosterone system and increase in sympathetic nervous system activity produce venous and arterial vasoconstriction. Excessive increases in preload and afterload cause increased energy expenditure for an already failing heart. Venodilators provide an increase in venous capacitance and decrease ventricular filling pressures, wall stress and preload. Arterial vasodilators provide reduction in afterload, increasing forward stroke volume. Most vasodilators exhibit activity on both arterial and venous vascular beds, although some vasodilators show specificity for certain segments of the vasculature.1
ANGIOTENSIN-CONVERTING ENZYME INHIBITORS
Mechanism of action
The renin–angiotensin system participates in short- and long-term control of blood pressure, as described in Chapter 2, through production of angiotensin II and aldosterone. Angiotensin II activates AT1 receptors on vascular smooth muscle cells of the precapillary arterioles and postcapillary venules causing vasoconstriction2 and inactivates bradykinin, a potent vasodilator. It also increases sympathetic tone and vasoconstriction by increasing release of adrenaline and decreasing its reuptake. Angiotensin II opens calcium channels in cardiac myocytes increasing cardiac contractility and increases heart rate by enhancing noradrenergic transmission and facilitating sympathetic tone.2 Angiotensin II regulates the tone of the efferent arteries of the kidney increasing the filtration fraction and resorption of sodium and water. It also acts directly on the renal tubular epithelial cells to inhibit excretion of sodium and water2 and acts directly on the adrenal cortex to produce aldosterone.
Angiotensin-converting enzyme inhibitors fall into three categories: (1) those containing a sulfhydryl group that binds to the zinc moiety of angiotensin-converting enzyme (captopril); (2) those containing a carboxyl group that binds to the zinc moiety of angiotensin-converting enzyme (enalapril, lisinopril); and (3) those containing a phosphinic acid group that binds to the zinc moiety of angiotensin-converting enzyme.3 All angiotensin-converting enzyme inhibitors prevent the conversion of angiotensin I to angiotensin II thereby attenuating the action of angiotensin II. They not only produce vasodilation by decreasing available angiotensin II but by increasing available bradykinin. As a result they inhibit the release of aldosterone from the adrenal cortex and decrease the sodium and water absorption from the kidney, potentiating the effects of diuretics. Angiotensin-converting enzyme inhibitors decrease the heightened stimulation of the sympathetic nervous system decreasing heart rate and vasoconstriction. Pharmacokinetics of angiotensin-converting enzyme inhibitors have undergone preliminary investigation; enalapril is poorly absorbed4,5 and effective doses have not been established.5 More recently quinapril has been evaluated in clinical cases using dosages extrapolated from other species and demonstrated changes in stroke volume and cardiac output which may suggest that further investigation of this drug is warranted.6 ( AF, IE, VMD)
Adverse effects
By reducing concentrations of angiotensin II, angiotensin-converting enzyme inhibitors decrease the kidney’s ability to autoregulate glomerular filtration fraction. In patients being treated with renin–angiotensin antagonists with marginal cardiac output, low blood pressure or concomitant use of Na+K+2Cl symport inhibitors, the glomerular filtration fraction may decline and an increase in serum creatinine concentrations may ensue.7 A significant decline in blood pressure may occur after an initial dose of angiotensin-converting enzyme inhibitors necessitating initiation of the drug with a low dose. Cough may develop weeks to months after initiation of therapy.1 Angioedema is a rare side effect in human patients.
HYDRALAZINE
The mechanism of action of hydralazine is poorly understood. Hydralazine reduces afterload by reducing systemic and pulmonary vascular resistance resulting in increase in forward stroke volume and a decrease in ventricular wall stress.3 Hydralazine has a direct positive inotropic activity on cardiac myocytes. It has little effect on venous resistance. In human medicine it is combined with an agent with vasodilating capacity such as organic nitrates (isosorbide dinitrate),3 although these increase the occurrence of side effects such as headache and dizziness in humans. Hydralazine increases renal perfusion by reducing renal vasculature resistance. Hydralazine is useful in patients with marginal renal function that cannot tolerate an angiotensin-converting enzyme inhibitor. Hydralazine is metabolized by the liver. It is available in oral tablets. The recommended dosage in the horse is 0.5–1.5 mg/kg every 12 hours orally.8
Diuretics
Diuretics are used in the treatment of heart failure to combat water and sodium retention by the kidneys. Diuretics reduce intravascular volume, ventricular filling pressures (preload) and wall stress. Although they usually do not cause a significant increase in cardiac output, diuretics do ameliorate the symptoms of congestive heart failure and may decrease the rate of progression of cardiac chamber dilatation by reducing ventricular filling pressure.3 Diuretics should be used intermittently as needed because of the acid-base and electrolyte disturbances that can be attributed to chronic diuretic therapy.
Inhibitors of the Na+K+2Cl symport
Inhibitors of an ion transport protein, the Na+K+2Cl symport, in the thick ascending limb of the loop of Henle were previously known as loop diuretics. They are the only diuretics that are effective alone in moderate to advanced heart failure. The other diuretics act more distally and are less effective because of the enhanced water and solute resorption in proximal nephron segments in heart failure. The Na+K+2Cl symport inhibitors may need to be combined with other diuretics in advanced stages of heart failure.3 Evidence suggests the Na+K+2Cl symport inhibitors bind to the chloride binding site but the exact mechanism is unknown.9 Inhibition of the symporter prevents the renal epithelial cell from absorbing sodium and chloride. Lack of ions in the medullary interstitium reduces its tonicity and decreases the driving force for water resorption in the collecting duct. Excessive sodium and fluid in the distal nephron enhances the secretion of potassium and hydrogen ions. Loop diuretics also inhibit absorption of calcium and magnesium because they are linked to sodium and chloride transport. Frusemide is the most widely used diuretic in this category. Effects of frusemide on the cardiovascular system have been well documented in the normal horse. Decrease in plasma volume, right atrial pressure, stroke volume and cardiac output and an increase in systemic vascular resistance occur acutely following administration. These rapid haemodynamic actions of loop diuretics are attenuated in studied human patients with chronic congestive heart failure.3 Chronic use of frusemide may induce hypokalaemia, hypochloraemic alkalosis, hyponatraemia and hypomagnesaemia. These electrolyte disturbances increase the risk for development of digoxin toxicity in patients treated with both drugs. Frusemide should be used intermittently to control the symptoms of heart failure. Horses treated with long-term digoxin and frusemide should have serum electrolyte concentrations monitored routinely. Supplementation with the appropriate electrolyte or change of diuretic is warranted if electrolyte disturbances are detected. The dosage of frusemide in the horse is 1 mg/kg IV, IM as needed, usually twice daily.10 Continuous rate infusions have been evaluated in the horse and cause more profound diuresis in the first 8 hours of treatment using a loading dose of 0.12 mg/kg followed by an infusion of 0.12 mg/kg/hour.11 This may be useful for initial stabilization of horses presenting with acute congestive heart failure, although there is no improvement in diuresis after the first 8 hours of treatment over conventional intravenous medication every 8 hours.11 The oral bioavailability of frusemide is poor and highly variable12 and therefore intramuscular administration is recommended for ongoing therapy. ( PH, VMD)
Potassium-sparing diuretics
Potassium-sparing diuretics are divided into two categories based on their molecular pharmacology. Diuretics, such as amiloride, inhibit sodium selective channels in the late distal convoluted tubule and the cortical collecting duct. The other category of potassium sparing diuretics are inhibitors of type I mineralocorticoid/glucocorticoid receptors and act as an aldosterone antagonist, such as spironolactone. Neither of these diuretics is sufficient alone in advanced heart failure although they may affect cardiac remodelling as discussed in Chapter 2. Care should be taken when using these diuretics with ACE inhibitors or in patients with reduced renal function, which may increase the serum potassium concentration. Potassium-sparing diuretics also cause less magnesium wasting than the Na+K+2Cl symport inhibitors. Pharmacokinetics and dosage for the potassium-sparing diuretics have not been established in the horse.
Cardiac glycosides(
AF, VT)
Cardiac glycosides occur naturally in a variety of plants and as venom in some toad species. Digitalis glycosides are those cardiac glycosides that are derived from Digitalis species of plants. Digitalis species include Digitalis purpurea (foxglove plant), and Digitalis lanata (digitoxin and digoxin).1 Cardiac glycosides have a chemical structure consisting of a steroid nucleus with an unsaturated lactone at the C17 position and one or more glycosidic residues at C3.1 Digoxin is the most commonly used cardiac glycoside in the horse. It is relatively inexpensive, easy to administer and the pharmacokinetics have been established. Pharmacokinetics and dosage have not been established for digitoxin in the horse.
Mechanism of action
Positive inotropic effect, inhibition of Na+K+ATPase
Depolarization of the cardiac myocyte occurs when fast sodium channels open producing phase 0 of the action potential.13 The depolarization of the cell activates L-type calcium channels which are voltage sensitive and found on the cell membrane.1,3 Calcium enters the cell producing the slow inward current, phase 2 of the cardiac action potential. This influx of calcium into the cell causes a release of calcium from the sarcoplasmic reticulum, an intracellular compartment. As the myocyte undergoes repolarization and relaxation, calcium is removed from the cell by a Ca2+ATPase that sequesters the calcium back into the sarcoplasmic reticulum. Also, a Ca2+ATPase and a Na+Ca2+ exchanger in the sarcolemmal membrane remove calcium from the cytoplasm.1,3 The calcium within the cell is exchanged for the sodium outside the cell. The difference in sodium concentration from inside the cell to outside the cell drives the Na+Ca2+ exchanger; if the sodium concentration within the cell is high, the exchange of calcium for sodium will be diminished.
Normally there is a large concentration gradient of sodium, most being outside the cell, which is maintained by the Na+K+ATPase pump exchanging intracellular sodium for extracellular potassium. Cardiac glycosides bind to and inhibit the Na+K+ATPase causing sodium to accumulate inside the cell. The increased amount of sodium inside the cell prevents the Na+Ca2+ exchanger from working efficiently and calcium is not pumped from the cell. The extra calcium within the cell is then taken up by the sarcoplasmic reticulum. This allows for more calcium to be released from the sarcoplasmic reticulum during contraction. The excess calcium binds to the sarcomere and increases the velocity and extent of sarcomere shortening increasing contractility.3 The alpha subunit of the Na+K+ATPase, the binding site for cardiac glycosides, is present on the extracytoplasmic side of the cell membrane.3 Excessive extracellular potassium decreases the binding affinity of cardiac glycosides for Na+K+ATPase by promoting dephosphorylation which is an initial step in the translocation of the enzyme into the cytosol.1 Therefore, increased extracellular potassium will reverse some manifestations of digitalis toxicity.
Regulation of sympathetic nervous system activity
In human patients in heart failure, an increase in sympathetic nervous activity may in part be due to insensitivity to the arterial baroreflex.1 The rise in blood pressure does not cause a decrease in sympathetic tone as it would in the normal heart. This insensitivity also contributes to sustained levels of noradrenaline, renin and vasopressin.1 Cardiac glycosides appear to decrease sympathetic nervous system activity by a centrally mediated effect and enhance baroreceptor sensitivity in patients with heart failure. Ferguson et al14 demonstrated in patients with moderate to severe heart failure given a cardiac glycoside, a decrease in heart rate and increased forearm blood flow and cardiac index. In addition, a simultaneous decrease in skeletal muscle sympathetic nervous system activity occurred, suggesting an overall decrease in centrally mediated sympathetic activity. The decrease in sympathetic nervous system activity and the increase in vagal tone will decrease automaticity and increase the diastolic resting membrane potential in the atrial and atrioventricular nodal tissue. The effective refractory period is prolonged and atrioventricular nodal conduction velocity is decreased.1 Because myocardial perfusion occurs in diastole, a decrease in heart rate will decrease myocardial ischaemia. A decrease in heart rate will also improve stroke volume by allowing more time for ventricular filling, and decrease myocardial oxygen consumption.
Pharmacokinetics
Digoxin is available as oral tablets, elixir and intravenous formulations. Because there is considerable variability in the systemic availability of oral formulations of digoxin on the market, the same product should be used consistently during maintenance therapy.3 Digoxin should not be given intramuscularly as it causes local irritation and is absorbed erratically.3 Pharmacokinetic studies of digoxin have been performed in the normal horse and in horses in congestive heart failure.15–20 Digoxin is eliminated by glomerular filtration and tubular secretion.3 In patients with decreased kidney function or those on diuretics or sympathomimetic agents with increased renal clearance, the dosage of the drug should be adjusted. Because of its large volume of distribution, digoxin cannot be removed by peritoneal dialysis.3 Digoxin is stored in the skeletal muscle, not fat, so dosage should be calculated on lean body mass. Digoxin has been shown to cross the placenta in humans.3 In the horse, 20–40% of digoxin in the plasma is protein bound.15
Bioavailability of oral digoxin is approximately 20%.17,19,20 Time to peak serum concentration is 1–2 hours after oral administration.16,19,20 A second rise in serum concentration occurs 4–8 hours later most likely due to enterohepatic recycling of the drug, slowed absorption or delayed availability of partially dissolved tablets.17,20 There is considerable variation in the serum concentration of digoxin following oral administration in individual animals.17,19 The reported range for the biological half-life of digoxin in the horse is 17–23 hours.15,17,19 Without loading doses, the serum concentration of digoxin reaches a steady state in the horse within 3.5 days for 12 hour dosing interval.19 The dosage for intravenous maintenance is 2.2 µg/kg every 12 hours and for oral maintenance is 11 µg/kg every 12 hours.20 An initial maintenance dose (2.2 µg/kg IV) given intravenously will result in achievement of therapeutic concentration more rapidly than oral administration for a portion of the dosing interval. Larger loading doses will increase the chance of toxicity.20 The therapeutic plasma concentration of digoxin is between 0.5 and 2.0 ng/mL.17
Therapeutic drug monitoring and adverse effects(
VT)
Because there is a narrow safety margin between the therapeutic and toxic levels of digoxin and because digoxin interacts with many commonly used medications, digitalis toxicity may occur during treatment. The initial signs of digoxin toxicity are anorexia, colic and diarrhoea due to alterations in sympathetic tone. Cardiac dysrhythmias are a common and potentially life-threatening result of digitalis intoxication. Close monitoring of the clinical response and plasma digoxin concentration must be performed in treated horses to avoid toxicity. The level at which clinical symptoms of toxicity show a rapid increase in prevalence is above 2.0 ng/mL. Some patients may show signs of toxicity within the “therapeutic” range. Serum levels should be taken 3.5 days after initiation of therapy since individual animals vary in their response.19,20 Serum samples should be taken at the time of peak and trough concentrations, 2 and 12 hours, respectively, after the last dose is administered.20
There are many drugs that may potentially interact with digoxin; H2 blockers, bran and albuterol may decrease the blood concentration of digoxin1 while propafenone, quinidine, verapamil, erythromycin, omeprazole, phenylbutazone and tetracycline may increase serum concentrations of digoxin.1,10 Hypoproteinaemia may decrease protein binding thereby increasing the unbound, metabolically active, concentration of the drug. Hypokalaemia, hypomagnesaemia and hypocalcaemia make the patient more sensitive to the toxic effects of digoxin. Hyperkalaemia decreases the binding affinity for digoxin and decreases the sensitivity to toxic effects, although it may exacerbate digoxin-induced conduction disorders and result in high-grade atrioventricular nodal block.3
Digoxin toxicity produces an increase in sympathetic tone and progressive inhibition of the Na+K+ATPase. The inhibition of Na+K+ATPase leads to a loss of cardiac cellular potential and excessive accumulation of intracellular calcium that alters cardiac conduction.10 Ectopic beats of atrioventricular junctional or ventricular origin, first-degree atrioventricular block and an excessively slow ventricular response to atrial fibrillation are the most common dysrhythmias in humans.1
In the case of digoxin toxicity, digoxin therapy should be immediately discontinued. Serum electrolyte and digoxin concentrations and acid-base status should be determined. Treatment for fluid, electrolyte and acid-base disturbances should be instituted. Even if the serum concentration of potassium is in the normal range, potassium can be administered to animals with life-threatening dysrhythmias, unless high-degree atrioventricular block is present. Hypomagnesaemia, hypokalaemia and hypocalcaemia make the patient more susceptible to toxic effects and should be corrected. Lignocaine or phenytoin may be used for worsening ventricular dysrhythmias that cause the patient to be haemodynamically unstable.21 Care should be taken with quinidine administration as it will increase unbound digoxin levels. If symptomatic, sinus bradycardia, sinoatrial arrest and 3rd degree atrioventricular block usually respond to the administration of atropine.1 Antidigoxin immunotherapy is available for humans, but is prohibitively expensive for equine patients.
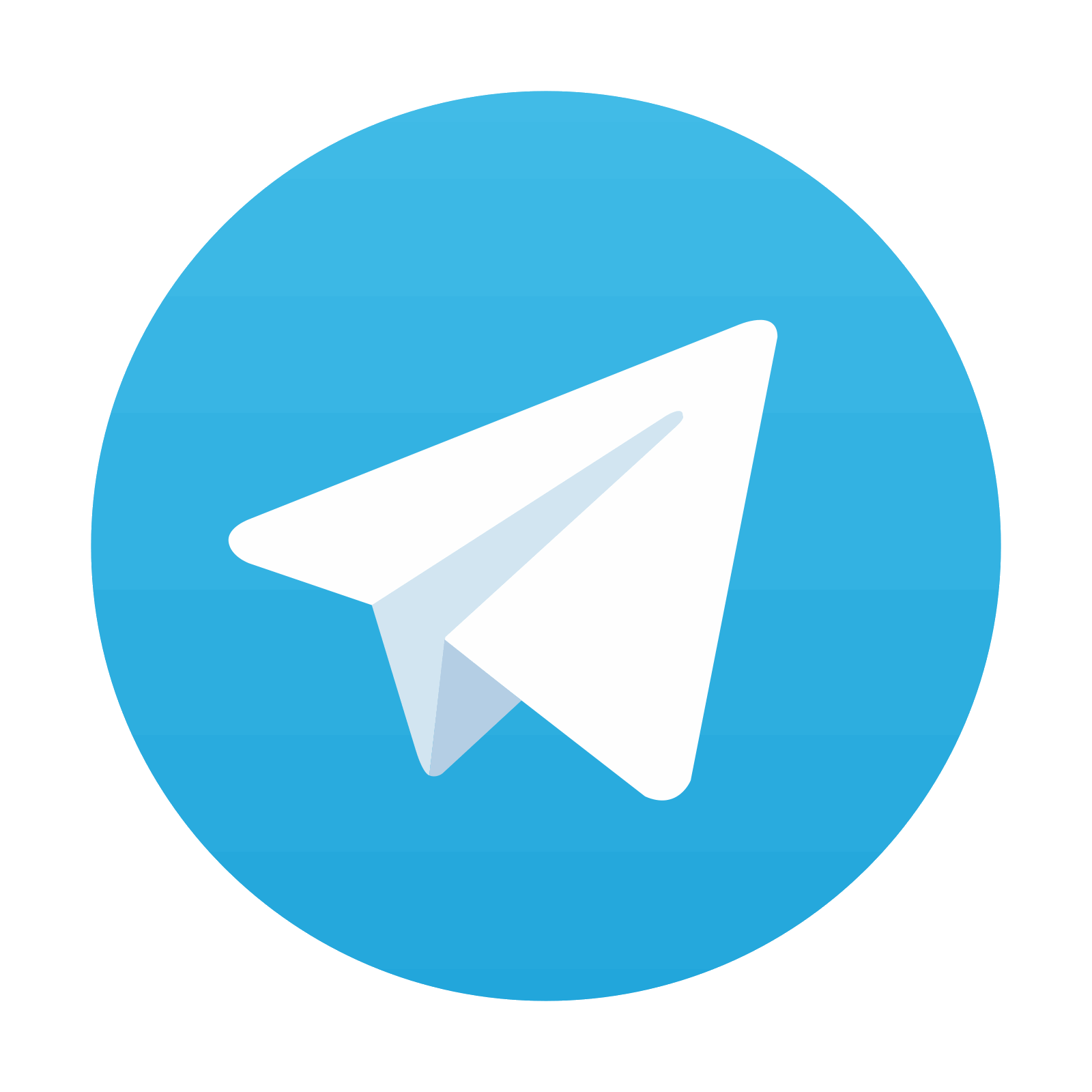
Stay updated, free articles. Join our Telegram channel
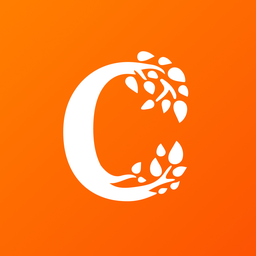
Full access? Get Clinical Tree
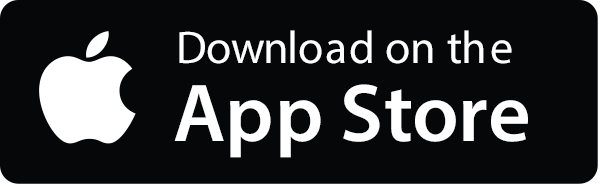
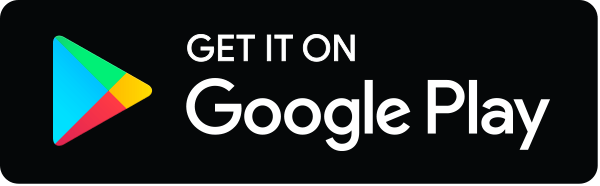