Marina Harper Thomas R. Smallman and John D. Boyce Bacteria from the genus Pasteurella are common mucosal commensals present in the upper respiratory tract (URT) of a range of animals. However, many of these organisms can also act as primary or secondary pathogens. The type strain of the genus, and the species most often identified as a primary pathogen, is Pasteurella multocida. The name “multocida” means “many killing” and this species is indeed a pathogen of many animal species with disease presentations that include hemorrhagic septicemia (HS) in ungulates, fowl cholera (FC) in birds, progressive atrophic rhinitis (AR) in pigs and rabbits, upper (URT) and lower (LRT) respiratory tract infections, and bite wound‐associated local and systemic infections in many species, including humans (Wilson and Ho 2013). P. multocida infections have a significant economic and ecological impact. Many hosts are important livestock animals and waterfowl are especially susceptible to large, fatal outbreaks of disease (Wobeser 1992). Outbreaks also regularly occur in wild ungulate species, including mass die‐off of saiga antelope due to HS (Kock et al. 2018). Recently P. multocida caused an outbreak of disease that killed thousands of wild rodents in southwestern China (Du et al., 2020). Organisms belonging to the genus Pasteurella are Gram‐negative, non‐spore‐forming, coccobacillus‐shaped bacteria (0.3–1.0 μm in diameter, 1.0–2.0 μm in length) that are facultatively anaerobic and catalase‐ and oxidase‐positive. Optimum growth temperature is generally considered to be 37°C, although many strains grow well at 40°C (the approximate body temperature of birds). They are unable to use citrate and all but Pasteurella oralis are unable to ferment lactose. Colonies are non‐hemolytic and generally pale gray in color with a characteristic “mousy” odor. Many P. multocida strains are capsulated and produce mucoid colonies that have a “blue hue” halo when illuminated with oblique light. Taxonomic revision of the Pasteurellaceae family is in progress. Currently, there are 13 validly named species within the genus Pasteurella (Table 10.1). However, 16S rRNA alignments and whole proteome analyses have defined Pasteurella sensu stricto as Pasteurella canis, Pasteurella dagmatis, P. oralis, Pasteurella stomatis, and P. multocida, which has three recognized subspecies, multocida, gallicida, and septica (Christensen and Bisgaard 2018). P. multocida strains can be further differentiated based on their capsule and lipopolysaccharide (LPS) surface antigens. Classically, strains have been typed using serological methods that identify these polysaccharides, but now multiplex polymerase chain reactions (mPCR) are available that target unique genes in the capsule and LPS biosynthetic loci (Townsend et al. 2001; Harper and Boyce 2017). Five different capsule serogroups (A, B, D, E, and F) are known to correlate with five distinct structures. Heddleston LPS serotyping recognizes 16 serologically distinct types (Heddleston et al. 1972), but the species is known to produce many more differentiable LPS structures (> 20). There are a total of eight unique LPS biosynthetic loci (L1–L8) that collectively are responsible for all known P. multocida LPS structures (Harper and Boyce 2017). Table 10.1 Pasteurella species, hosts, diseases, or location. Analysis of the 16S rRNA sequences obtained from other current members of the genus suggests that [Pasteurella] aerogenes and [Pasteurella] mairii are the most closely related to, but clearly separate from, the species within Pasteurella sensu stricto. In contrast, [Pasteurella] bettyae and [Pasteurella] caballi cluster close to Bibersteinia trehalosi; [Pasteurella] caecimuris clusters with Rodentibacter spp.; [Pasteurella] langaaensis clusters close to Gallibacterium spp.; [Pasteurella] skyensis clusters with Cavibacterium pharyngocola, and [Pasteurella] testudinis clusters with Chelonobacter oris. All five species within Pasteurella sensu stricto can cause disease. However, this chapter focuses on P. multocida, as this species is the most problematic with respect to disease in production animals and has been the most widely studied (Table 10.1). The genus Pasteurella lies within the family Pasteurellaceae, which contains several other genera that contain human and animal pathogens, including Haemophilus, Actinobacillus, Aggregatibacter, Avibacterium, and Mannheimia. A comparison of the first complete P. multocida genome (strain Pm70) with genomes representing Haemophilus influenzae and Escherichia coli, all within the Gammaproteobacteria, indicated that species within the Pasteurella diverged from Haemophilus spp. approximately 270 million years ago and from Escherichia spp. and the Enterobacteriaceae family approximately 680 million years ago (May et al. 2001). Pasteurella spp. reside harmlessly in the URT and gastrointestinal tracts of many birds, mammals, and reptiles. P. multocida has been isolated from healthy dogs and cats, waterfowl, mammals (including marsupials and marine mammals), and reptiles, including Komodo dragons (Wilson and Ho 2013). P. multocida can also be isolated from healthy livestock animals including chickens, turkeys, cattle, swine, sheep, and goats. Carriage rates vary widely between species and between subpopulations within species, with many individuals having no evidence of P. multocida carriage. Animals harboring P. multocida can act as a reservoir for transmission to naïve animals. P. multocida within saliva and respiratory secretions can be inhaled or introduced into bites or open wounds (Wilson and Ho 2013). The bacterium may also be introduced into the URT of naïve animals by direct contact with contaminated feces or carcasses. P. multocida can also contaminate soil and water sources and levels of the bacterium can be very high during large outbreaks of disease. However, these environmental niches provide only a temporary reservoir for P. multocida (Blanchong et al. 2006). Healthy carriers of P. multocida are predicted to shed the bacterium only intermittently from the URT, unless the immune system of the carrier is compromised, as demonstrated by dexamethasone treatment of carrier buffalo which induced shedding of P. multocida from the URT and ureters (De Alwis 1992). Overcrowding and environmental stressors have also been associated with increased transmission and carriage of P. multocida. Large outbreaks of HS in saiga antelope are associated with periods of unusually high temperatures and humidity (Kock et al. 2018). A high population density is also an important contributor to P. multocida transmission and disease, as can occur during live export shipping, in feedlots, and in commercial poultry farms. P. multocida has the exceptional ability to cause disease with multiple presentations in a wide range of animal species. Toxigenic P. multocida are a unique subset of strains that produce a toxin called PMT (Pasteurella multocida Toxin), which is the causative agent of AR in pigs and rabbits. All other disease presentations are typically caused by P. multocida strains that do not produce toxin. These include FC in birds, HS in ungulates, and a range of respiratory disease in other animals (Wilkie et al. 2012). Diseases range from self‐limiting/chronic URT infections, which have little effect on the overall health of the host, to acute LRT or systemic infections that can cause severe morbidity or be rapidly fatal. P. multocida can also be part of multispecies infections that include other bacteria and/or respiratory viruses. Disease presentation and host range in some instances correlates with specific surface carbohydrates. For example, porcine AR isolates typically produce a serogroup D capsule and bovine HS isolates all belong to LPS genotype L2 and produce a serogroup B capsule or the closely related serogroup E capsule. FC can present as an acute or chronic disease in all bird species but is most problematic in poultry and waterfowl. Avian cholera isolates typically belong to capsule serogroup A, F, or D (Smith et al. 2021). In both chronic and acute FC, clinical signs include ruffled feathers, lethargy, depression, fever, sneezing, and nasal discharge. Acute FC is typically of short duration (one to three days) and can be fatal before any signs of disease become apparent (Wilkie et al. 2012). In chronic FC, swelling of wattles and combs can occur but after approximately 14 days of disease clinical signs start to reduce in severity. However, the bacterium can persist in the URT, LRT, liver, and lymphatic system, providing a reservoir for future outbreaks of disease (Mbuthia et al. 2011). The severity of FC disease can vary greatly and is dependent on the infecting strain, infectious dose, host species, and individual immune response. Turkeys, pheasants, and partridges inoculated with the same virulent FC strain were reported to be more susceptible than chickens (Petersen et al. 2001). A strong immune response, led by the recruitment of heterophils, can lead to hemorrhage and necrosis in birds infected with P. multocida (Bojesen et al. 2004; Mbuthia et al. 2011). HS is an acute systemic disease that affects buffalo, cattle, and wild populations of ungulates in regions of Africa and Asia. Large outbreaks in Asia cause significant livestock losses each year (De Alwis 1992). P. multocida strains that cause HS exclusively belong to the closely related serogroups B and E (Asian and African isolates, respectively) and LPS genotype L2 (Harper and Boyce 2017). The URT of susceptible animals is colonized before the bacterium invades the mucosa at the tonsils and migrates to nearby lymph nodes, most likely inside macrophages (Annas et al. 2014). P. multocida then disseminates throughout the host, causing an overwhelming systemic infection and death. Peracute HS infections develop rapidly (4–12 hours) and death can occur suddenly without prior signs of disease (De Alwis 1992). The lipid A component of LPS, known as endotoxin, is the main driver of severe septic shock observed in peracute cases of HS. In acute HS, the levels of endotoxin rise more slowly, and the disease can last up to five days (Horadagoda et al. 2001). Animals will have a fever, depression/apathy, loss of appetite, and nasal discharge. Late signs of infection include labored breathing, increased respiration rate, a mucopurulent nasal discharge, and swelling of salivary glands, the lower chest, and forelegs. Death is almost certain once the animal becomes recumbent and develops clear signs of septicemia and septic shock. Animals that have survived HS shed P. multocida and some become chronic carriers and may act as a source of future outbreaks (De Alwis 1992). AR is a chronic disease that primarily affects the nasal cavities of pigs and rabbits. Clinical signs of infection and turbinate damage include nasal discharge, sneezing, and snuffling (Wilkie et al. 2012). Mild, non‐progressive AR is caused by Bordetella bronchiseptica strains that produce dermonecrotic toxin (DNT). However, a more severe, progressive AR is caused by P. multocida strains that also produce a dermonecrotic toxin, called PMT, and typically belong to capsule serogroup D (Horiguchi 2012). AR starts with colonization of the host URT by toxigenic P. multocida strains, often facilitated by a pre‐existing infection with B. bronchiseptica. P. multocida AR strains colonize the tonsils and continually release PMT that modifies the activity of host G proteins resulting in widespread activation of signaling pathways (for more on PMT see below). In response to this perturbation, osteoclasts disproportionally increase in the nasal cavity, resulting in bone resorption, atrophy of the nasal turbinates, deviation of the nasal septum, and ultimately permanent distortion and twisting of the snout. Untreated animals have an increased chance of later developing LRT infections (Horiguchi 2012). P. multocida LRT infections can occur in many animal species and are especially common in cattle and other ungulates. Infections range from peracute/acute fulminating pneumonia observed in cattle, to more chronic and localized pneumonia often observed in pigs and sheep. Colonization and persistence of P. multocida in the lungs can occur alone, or more commonly together with a virus or another bacterial species such as Mannheimia haemolytica or Histophilus somni (Wilkie et al. 2012). Clinical signs of Pasteurella pneumonia include inflammation of the nasal mucosa, increased nasal secretions, sneezing, fever, labored breathing, and frothing at the mouth. In severe cases, death occurs from respiratory failure (Wilkie et al. 2012). Pasteurella species can cause a wide range of zoonotic infections in humans, often following a cat or dog bite. Most are caused by P. multocida capsule serogroup A strains, although serogroup F strains have also been isolated (Ujvari et al. 2019; Smith et al. 2021). Data from one extensive study on domestic animal bites found that P. multocida was present in 12% of infected dog‐bite wounds and 62% and infected cat‐bite wounds. Of the other Pasteurella species, P. canis, P. stomatis, and P. dagmatis were present in 13%, 6%, and 2% of infected dog bite wounds, and in 1.5%, 3%, and 5.3% of infected cat bite wounds, respectively (Talan et al. 1999). Edema, cellulitis, and purulent exudate are often present at the site of the wound infection and untreated the infection may lead to necrotizing fasciitis. Cases of bacteremia, meningitis, peritonitis, pneumonia, or septicemia caused by P. multocida have also been reported (Wilson and Ho 2013). Recent bioinformatic analysis has shown strains of P. multocida isolated from human infections share similarities with isolates from commensal strains isolated from domestic animals but are genetically distinct from strains that cause most animal infections, indicating possible human‐specific virulence factors (Smith et al. 2021; our unpublished data). The polysaccharide capsule is a primary virulence factor and can be used to classify strains using serology or mPCR into five serogroups, A, B, D, E, and F (Boyce et al. 2000). Capsules of type A, D, and F strains are closely related and are composed of hyaluronic acid, heparin, and chondroitin, respectively (Figure 10.1). These polymers are also found in the eukaryotic extracellular matrix and are predicted to “mask” the surface components of the bacterium from the host innate immune response. The serogroup B and E main chain disaccharide repeat units both consist of a mannosaminuronic acid derivative linked to an N‐acetylglucosamine but differ with respect to the linkage of the side‐branch fructose and the addition of glycine onto the serogroup B structure (Michael et al. 2021; Figure 10.1). Figure 10.1 Overview of Pasteurella multocida virulence factors and important cellular components. P. multocida strains express multiple adhesins including filamentous hemagglutinin (up to two), ComE1 (also binds DNA), OmpA and the tight adherence pilus (Tad) and the type IV pilus. Toxigenic strains of P. multocida release a toxin called PMT, which alters host cell signaling pathways through deamidation of heterotrimeric G proteins. Sialic acid (Neu5Ac) uptake from host sources, for catabolism or possible addition to surface structures such as lipopolysaccharide (LPS), requires surface sialidases (e.g. NanH and NanB) and a sialic acid transport system. Iron uptake is crucial for P. multocida in vivo survival; outer‐membrane receptors including HemR, HgbA, and HasR interact with the ExbB/ExbD/TonB transport system. The ferric uptake regulator (Fur) generally represses expression of certain outer membrane proteins in the presence of excess iron. Fur, Hfq (and associated sRNAs), and factor for inversion stimulation (Fis) are important regulators of virulence factors including capsule biosynthesis. Expression of capsule biosynthesis genes is downregulated in late exponential phase, probably due to reduced levels of Fis and activation of the stringent response, which increases cellular levels of the nucleotides guanosine pentaphosphate and guanosine tetraphosphate, collectively named (p)ppGpp. Both Fis and Hfq activate filamentous hemagglutinin expression and Hfq stimulates PlpE expression. Antibodies to LPS, OmpH and PlpE are protective. The capsule (blue) is composed of one of five polysaccharides, the A, B, D, E, and F structures representing the five different capsular serogroups are shown lower right. The serogroup A capsule biosynthetic pathway and associated genes are shown within the cell; UDP‐N‐Acetylglucosamine is also used for peptidoglycan synthesis and LPS biosynthesis (not shown). LPS (represented by black wavey lines on cell surface) is an important virulence factor and a strong stimulator of the host immune response. The LPS outer core structures produced by the 16 Heddleston LPS serotypes are structurally distinct (schematic of outer core structures shown upper right). Serotypes 2 and 5 LPS are serologically distinct but structurally they differ only by the presence/absence of a phosphoethanolamine on an inner core sugar (not shown). Additional LPS outer core structures have now been identified and collectively all are generated from only eight distinct LPS biosynthetic loci (L1 to L8; top right). Truncated outer core structures typically occur by way of mutations in sugar transferase gene/s within a particular LPS locus that result in arrest of LPS assembly. Sugars present in capsule and LPS structures are; glucose (blue circle); galactose (yellow circle); fructose (dark red pentagon); heptose (pink hexagon); rhamnose (grey triangle); N-acetyl-galactosamine (yellow square); N-acetyl-glucosamine (blue square); phosphocholine (red hexagon); phosphoethanolamine (black hexagon); (1S)-2-acetamido-2-deoxy-D-galactose (Yellow rectangle); 1-((4-aminobutyl)amino)-3-hydroxy-1-oxopropan-2-yl hydrogen phosphate (pink rectangle); 3-acetamido-3,6-dideoxy-α-D-glucose (green square); glucuronic acid (white and blue triangle); mannosaminuronic acid (green and blue trangle). Figure 10.2 Schematic representation of the capsular polysaccharide biosynthesis loci representing serogroups A, D, F, B, and E (left) and structures of the corresponding polysaccharides (right). Genes of the same color encode proteins with > 90% amino acid identity, genes of the same shade of gray encode proteins with between 70% and 89% identity, and unfilled genes encode proteins with < 70% identity. Horizontal lines below each locus indicate the position of the amplicons produced using the capsule type‐specific multiplex polymerase chain reaction (Townsend et al. 2001). Vertical dotted lines separate the loci into regions involved in ATP‐dependent capsule export (region 1), type‐specific polysaccharide biosynthesis (region 2) and lipidation and membrane anchoring (region 3, shaded gray). Note that in serogroups B and E the lipA gene (encoding a PhyA homolog and predicted to be a region 3 lipidation gene) is contiguous with the region 1 genes. Sugar abbreviations; GlcNAc, N‐acetyl‐glucosamine; GlcA, Glucuronic acid, GalNac; N‐acetyl‐galactosamine; ManNAc, Mannosaminuronic acid; Gly, Glycine; Fru, Fructose; Fruf, Fructofuranose. Source: Based on Townsend et al. (2001). The P. multocida capsule biosynthesis loci are typical group II‐type capsule biosynthesis loci with three distinct regions (Boyce et al. 2000). Region 1 is highly conserved in all P. multocida capsule loci and contains the genes required for the ATP‐binding cassette (ABC) transport system responsible for exporting the polysaccharide to the bacterial surface. Region 2 genes differ between serogroups and encode proteins required for the synthesis of each type‐specific polysaccharide. This includes the specific synthase required to add the sugar monomers to the growing polymer. In serogroup A, D, and F, these synthases are HyaD, DcbF, and FcbD, respectively (Figure 10.2). The precise function of each gene within region 2 in serogroup B and E is currently unknown. Primers used in the capsule multiplex mPCR anneal to unique sequences within region 2 of each locus (Townsend et al. 2001). Region 3 genes encode proteins predicted to be required for the lipidation and membrane anchoring of the capsular polysaccharide (i.e. PhyA and PhyB in serogroups A, D, and F and LipA and LipB in serogroups B and E). The genes encoding these proteins are co‐located, except for the serogroup B and E lipA and lipB which flank the region 2 genes (Figure 10.2; Townsend et al. 2001). The first definitive evidence that P. multocida capsule was a major virulence factor came from experiments that showed a cexA capsule export mutant was strongly attenuated for virulence in mice compared with the highly virulent serogroup B parent strain M1404. Murine peritoneal macrophages could also readily phagocytose the M1404 cexA mutant but not the wild‐type parent (Boyce and Adler 2000). Similar experiments using the serogroup A FC isolate X‐73 and an isogenic capsule (hexA) mutant showed the capsule mutant was strongly attenuated for disease in both mice and chickens and was highly sensitive to complement‐mediated killing (Chung et al. 2001). Taken together, these data support the critical role of capsule in virulence and avoidance of the host innate immune response. Like most Gram‐negative bacteria, the external surface of the P. multocida outer membrane is predominantly comprised of LPS molecules, the lipid A component of which is a potent endotoxin (Horadagoda et al. 2001). Each LPS molecule consists of a hydrophobic lipid A membrane anchor, a conserved inner core oligosaccharide, and a highly variable outer core oligosaccharide. The lipid A structure of the LPS from a P. multocida HS isolate has recently been reported (Tawab et al. 2020) but it is not known whether this structure is conserved across the species. The complete repertoire of inner and outer core oligosaccharide structures produced by P. multocida has also been determined (Harper and Boyce 2017; Figure 10.1). Strains are classified into eight LPS genotypes (L1 to L8) using mPCR analysis with primers that anneal to unique regions within each LPS outer core biosynthetic locus (Harper and Boyce 2017). Each outer core locus contains a range of genes, the majority of which encode sugar transferases, each responsible for the transfer of a specific sugar to a specific position on the LPS structure (Harper and Boyce 2017). The LPS produced by each P. multocida strain initiates a specific antibody response that historically was used to serologically classify strains into 16 Heddleston LPS serotypes (Heddleston et al. 1972). However, many more LPS types exist due to the existence of truncated LPS structures in addition to the full‐length “parent” structures. Truncated LPS structures typically result from inactivating mutations within sugar transferase genes, resulting in the arrest of LPS assembly and truncation of the full‐length structure (Harper and Boyce 2017). Virulence studies have shown that a full‐length LPS structure is required for the P. multocida A:L1 strain VP161 to cause systemic disease in chickens. However, some VP161 mutants expressing truncated LPS molecules can persist at the site of intramuscular injection but not in the blood (Harper and Boyce 2017). Many isolates recovered from natural infections have mutations within the LPS loci and express shortened LPS structures, suggesting that strains lacking full‐length LPS may reside naturally in tissues other than blood, such as the tonsils or other niches within the URT. Interestingly, some LPS mutants are highly attenuated for systemic disease in chickens but can still cause systemic disease in mice, demonstrating that different animal species respond differently to this important component of the P. multocida cell surface (Harper and Boyce 2017). The P. multocida dermonecrotic toxin PMT is encoded by toxA, a gene located within a lysogenic bacteriophage that is integrated into the genome of P. multocida AR isolates that typically belong to capsular serogroup D or A (Pullinger et al. 2004). The toxin is passively released from P. multocida cells colonizing the URT, especially within the turbinates. It belongs to the A‐B family of toxins; the B domain facilitates binding to the host cells while the A domain exerts a toxic effect on the heterotrimeric G class of GTPases following transport of the toxin into the cytosol (Wilson and Ho 2012). The eukaryotic heterotrimeric GTPases regulate the amount of available GTP to control multiple cell‐signaling pathways. In the inactive state, the G protein complex consists of GDP‐bound alpha, beta, and gamma subunits. When GTP displaces GDP, the trimer dissociates into two active effectors, GTP‐bound alpha G protein and the beta/gamma complex (Wilson and Ho 2012). At the molecular level, PMT deamidates a specific glutamine on the alpha subunit of the G protein subunits Gαq, Gαi, and Gα12/13 (Orth et al. 2013). This stops the conversion of the active GTP‐bound Gα protein to the GDP‐bound inactive state, which subsequently results in accumulation of the active GTP‐Gα protein and the beta/gamma G protein complex. The abundance of activated Gα protein affects many cell‐signaling pathways and results in increased levels of inflammatory cytokines. One of the most profound effects of PMT is the proliferation of osteoclasts, responsible for bone remodeling/degradation and resorption, and a deficiency of bone‐forming osteoblasts (Wilson and Ho 2012). This imbalance leads to the distortion of the nasal turbinate bones, as observed in animals with AR disease. PMT permanently activates the Gαq proteins, which ultimately affects the expression of genes involved in osteoblast formation (Siegert et al. 2013). Normal osteoclastogenesis is also affected. Unlike the normal process, PMT‐induced osteoclast formation is receptor activator of nuclear factor κB ligand‐independent and leads to an increase in the master transcription factor that controls osteoclast differentiation (Chakraborty et al. 2017). Osteocytes (derived from osteoblasts) are the main cells within mature bone and are also affected by the toxin (Heni et al. 2018). Thus, PMT exerts its effect on the cells within the nasal turbinates in a myriad of ways. Current data indicates the toxA
10
Pasteurella
Introduction
Characteristics of the Organism
Pathogenic Species
Species
Hosts
Disease or isolation site
References
Pasteurella sensu stricto
P. canis
Normal flora in dogs
Bite wound infections
(Mutters et al. 1985)
P. dagmatis
Normal flora in dogs
Bite wound infections
(Mutters et al. 1985)
P. multocida
Pathogen of many mammals and birds. Normal flora in cats, dogs and other species
Fowl cholera, hemorrhagic septicemia, atrophic rhinitis, respiratory infections, bite wound‐associated infections
(Rosenbusch and Merchant 1939)
P. oralis
Normal flora in cats, dogs, bats, and ferrets
Bite wound infections (limited reports)
(Christensen et al. 2012)
P. stomatis
Normal flora in dogs
Bite wound infections
(Mutters et al. 1985)
Current validly named members of the genus but not assigned to Pasteurella sensu stricto
[P.] aerogenes
Pigs, humans
Pig and hamster bite‐wounds, asymptomatic bacteriuria, swine abortion
(Christensen et al. 2005)
[P.] bettyae
Humans
Genitourinary infections and bacteremia
(Sneath and Stevens 1990)
[P.] caballi
Horses, pigs, humans (horse‐bites)
Respiratory infections and bite wound infections
(Schlater et al. 1989)
[P.] caecimuris
Mice
Abscesses
(Lagkouvardos et al. 2016)
[P.] langaaensis
Chickens
Respiratory tract, possible commensal
(Mutters et al. 1985)
[P.] mairii
Pigs
Reproductive and intestinal tract of swine, spontaneous abortions
(Sneath and Stevens 1990; Christensen et al. 2005)
[P.] skyensis
Salmon
Gill infections, associated with significant mortality
(Birkbeck et al. 2002)
[P.] testudinis
Tortoises
Respiratory, oral, and reproductive tract; healthy and diseased animals
(Snipes and Biberstein 1982)
Source of Infection: Ecology, Evolution, and Epidemiology
Types of Disease and Pathologic Changes
Fowl Cholera
Hemorrhagic Septicemia
Atrophic Rhinitis
Lower Respiratory Tract Infections
Zoonotic Infections in Humans
Virulence Factors and Pathogenomics
Capsule
Lipopolysaccharide
Pasteurella multocida Toxin
Stay updated, free articles. Join our Telegram channel
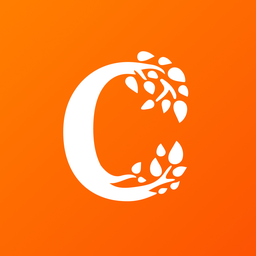
Full access? Get Clinical Tree
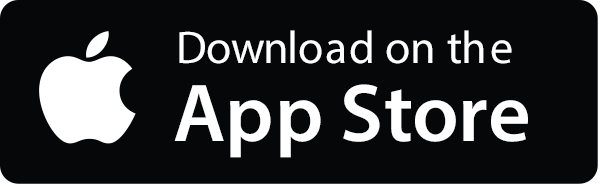
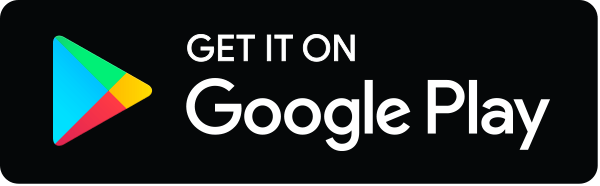