, Bryan D. Choi2 and Duane A. Mitchell3
(1)
Department of Pathology, Duke University Medical Center, Durham, NC, USA
(2)
Duke Brain Tumor Immunotherapy Program, Division of Neurosurgery, Department of Surgery and Department of Pathology, Duke University Medical Center, Durham, NC, USA
(3)
Duke Brain Tumor Immunotherapy Program, Division of Neurosurgery, Department of Surgery, The Preston Robert Tisch Brain Tumor Center, Duke University Medical Center, Durham, NC, USA
Abstract
We have demonstrated that dendritic cells (DCs) loaded with total tumor RNA (ttRNA) represent a potent platform for the induction of antitumor immunity in preclinical murine glioma models. DCs are potent immunostimulatory cells that represent a promising contemporary biologic entity for realizing the potentials of immunotherapy. The use of RNA to encode tumor antigens for DCs was pioneered at our institution by Drs. Eli Gilboa and Smita Nair, but the ability of RNA-loaded DCs to stimulate potent antitumor immunity has been independently confirmed in several murine and human systems. In fact, there is accumulating evidence that RNA transfection represents a superior method for loading antigens onto DCs. In this chapter, we describe the methods involved in using DCs electroporated with ttRNA to potentiate the host immune response against infiltrating MGs. Furthermore, this chapter includes an in-depth review of the history of immunotherapy for high-grade gliomas using animal models as well as an overview of some of the more compelling immunotherapeutic strategies known to date.
Key words
GliomasBrain tumorImmunotherapyDC vaccinesDendritic cells1 Introduction
1.1 Immunotherapy of Malignant Gliomas
The prognosis for patients with primary malignant gliomas (MGs) remains very poor despite aggressive multimodal therapy, including surgical resection and radiotherapy plus temozolomide (TMZ) (5). Moreover, the nonspecific nature of conventional therapy for MGs often results in incapacitating damage to normal brain (6–8). This nonselectivity calls for novel therapeutic strategies that can precisely target invasive tumor cells while minimizing collateral damage to nearby brain parenchyma. The rationale for employing the immune system to target MGs is based on the premise that the inherent biologic specificity of the immune system can meet the clear and urgent need for a more precise medical therapy. Furthermore, the bioavailability of the immune system’s cellular effector mechanisms and capacity to mount immunologic memory responses have been shown to be quite vast, even within the once-believed immunoprivileged CNS (9–12).
Cellular-based immunotherapy for MGs involves the ex vivo manipulation of cells in some fashion with the hopes of inducing an effective, specific, antitumor response upon transfer into the host. Cellular immunotherapy can be bifurcated into two modes of cell priming: (1) active immunotherapy, which involves vaccination of the host-modified tumor cells or with tumor antigen-loaded antigen presenting cells (APCs), or (2) adoptive immunotherapy, which utilizes the transfer of effector cells (such as natural killer cells or T cells) that are primed and expanded ex vivo and transferred back into the host to target the tumor (7). The generation of effective immunity against tumor-specific antigens (TSAs), has been shown, at least in preclinical models, to establish an immunologic repertoire of memory cells that can survey the host and prevent tumor recurrence, an additional benefit that no other targeted therapy possesses (13–15).
1.2 The Immunoprivileged Central Nervous System
The central nervous system (CNS) has previously been regarded as a site of limited immune surveillance. This concept is based on the primary studies of Medawar (16), Barker, and Billingham who demonstrated that allografts are tolerated better when implanted into the CNS compared to other tissues (17, 18). The “immunologic privilege” of the CNS has long been attributed to the blood–brain barrier (BBB) and the lack of a classic lymphatic system draining into the periphery (19).
However, over the past several years, the longstanding model that the CNS did not have the capability of interacting with activated lymphocytes, was void of specialized resident APCs, and lacked a lymphatic drainage system has been challenged. It is now accepted that lymphocytes traverse the BBB endothelial cells to ultimately reside in perivascular spaces or localize to the neuropil (20, 21). Moreover, it is also established that antigens efficiently drain into cervical lymph nodes via the cribroid plate and perineural sheath of cranial nerves (22, 23), and recent data suggest similar routes for APCs (24–26). Several studies in mice have supported the notion of a permissive barrier for immune cell trafficking. Adoptive transfer studies have revealed that T cell activation is sufficient to induce the migration of T cells into the CNS in the absence of an exogenous inflammatory stimulation of the endothelial barrier. One model suggested that inflammatory conditioning could induce endothelial cells to become competent in supporting cell trafficking by the induction of adhesion or chemoattractant molecules (27). In another study, transgenic GFAP-CCL21 mice with constitutively expressed CCL21 (a ligand that mediates lymphocyte homing to secondary lymphoid organs) on BBB endothelial cells facilitated the homeostatic CD4+ T cell proliferation of T cells isolated from lymph nodes draining the CNS. In another murine model, it was observed that CNS expression of CCL21 augmented the migration of CD4+ T cells from perivascular spaces into the CNS parenchyma of T. gondii infected mice (28). These findings contribute to the growing literature demonstrating that the immune privileged CNS can regulate T cell-mediated immunity.
Given the evidence for T cell trafficking into the CNS, the vast array of aberrantly expressed and mutated proteins present in tumor cells should permit them to be identified as foreign and ultimately rejected by the immune system. This concept of tumor surveillance as a normal function of the immune system has supporting evidence in that physiologic antitumor immune responses can often be detected in patients with cancer, although such responses are insufficient to prevent the progression of malignancy. Reasons for this insufficient response may be due to the debilitation of the immune system by locally and systemically-induced immunosuppression (29, 30). In a number of studies, MG cells have been shown to secrete a number of substances that suppress antitumor immunity, including TGF-β2, prostaglandin (PGE2), and IL-10 (31–33). Other mechanisms of immune evasion include production of extracellular matrix proteins, such as tenascin C and hyaluronic acid, disruption of the intracellular adhesion molecule (ICAM)-1, and downregulation of MHC class I antigens (34, 35).
1.3 Antigen Recognition and T Cell Trafficking Within the CNS
Microglia, which comprise about 10% of the total glial population in the CNS parenchyma (36), are a unique CNS cell population due to their mesodermic origin (as opposed to the neuroectodermally derived resident CNS cells). Having developed from a common precursor cell, microglia share many properties with macrophages (37, 38). Amidst virtually all inflammatory conditions in the CNS, parenchymal microglial cells rapidly upregulate MHC class II expression, suggesting that they may participate in antigen presentation to CD4+ T cells during the inflammatory process (38–42). Activated microglia also upregulate the co-stimulatory molecules CD40, CD80, and CD86, which render them capable for antigen presentation to T cells (21, 43–45). Furthermore, microglia have been shown to phagocytose myelin (46–48), indicating that these cells can activate myelin-specific CD4+ T cells. In preclinical models, microglia infected with Theiler’s murine encephalomyelitis virus are capable of efficiently processing and presenting not only endogenous viral epitopes but also exogenous myelin epitopes to CD4+ T cells (49).
Naive T cells are not inherently located within the CNS due to their inability to pass through the BBB in the context of homeostatic conditions (i.e. the lack of an acute inflammatory response). When T cells have been activated against neurotrophic pathogens or CNS autoantigens, however, they cross the BBB and are restimulated upon encounter with their respective antigen on target cells and local APCs (50, 51). Recent studies of murine brain tumor models have shown that the tumor microenvironment promotes the proliferation of tumor-specific T cells and differentiation into cells with enhanced effector function (52). While activated T cells are thought to patrol the CNS in an antigen-independent manner, cells that encounter their cognate antigen are retained for longer periods within the CNS than those that do not encounter target antigen in the CNS (52). Chemokine receptors and αβ-integrin receptors together provide a coordinated homing signal for mediating the destination of trafficking lymphocytes. One such chemokine receptor, CXCR4, has been demonstrated to mediate neural stem cell localization to MGs (53, 54), and we have demonstrated that CXCR2 expression in T cells provides enhanced trafficking in response to glioma-secreted chemokines (IL-8 and GRO-1α) in vitro and in vivo (55).
1.4 A History of Animal Models: Proof-of-Concept for Immunotherapy
Beginning with preliminary studies by Medawar (16) and Burnet (56), the concept of immunological surveillance of tumor cells was based on immunogenicity studies utilizing implanted (57), carcinogen-induced (58, 59), or virus-induced (59) tumors. Using these models, it was believed that a tumor cell can express the genes encoding proteins or “factors” that the immune system can recognize to discriminate between normal and tumor cells. In 1970, Stutman, who was one of the contenders of this theory (60), investigated tumor development in immunodeficient (athymic “nude”) mice that lacked adaptive immunity. Contrary to what was expected, the mice failed to show any notable acceleration in either induced carcinogenesis or growth of the implanted tumors. Thus, because of the absence of T and B cells in these mice, the conclusion was made that the immune system was not involved in tumor surveillance. It was later discovered that some immune surveillance components in nude mice, such as γδ T cells and natural killer (NK) cells, are fully functional.
Genetic recombination technologies employed in the 1990s allowed the generation of mouse lines in which the adaptive immune system was partially or entirely disrupted. These knockout lines allowed the role of adaptive immunity in protection against tumors to be more precisely analyzed. One model of particular utility was the generation of Recombination Activating Gene (RAG)-deficient murine model. These mice lack the ability to undergo somatic recombination, a process that is required for rearrangements of both immunoglobulin and T cell receptor genes to generate functional T and B cells (61). Another commonly used murine model with a disrupted immunity is the severe combined immunodeficient (SCID) model. Due to a mutation that inactivates the gene for the catalytic subunit of DNA-dependent protein kinase, this defect impairs DNA double-strand break repair and prohibits V(D)J recombination (62). Schreiber et al. (63) conducted experiments on spontaneous carcinogenesis with SCID and RAG−/− mice and with mice defective in signaling pathways involving IFN-γ, an important immunoregulatory cytokine for the formation of functional cytotoxic T lymphocytes (CTLs). An accelerated emergence of spontaneous and induced tumors was observed in these experiments as well as a shorter survival time. Within the field of tumor immunology, these findings from RAG−/− and SCID mice contributed to the revival of the immunological surveillance concept. It was also observed that when carcinogen-induced tumor cells were implanted into recipient mice, the tumor could be cleared in only a few recipients, indicating that some mice were incapable of effective immunological surveillance. From these findings, it was evident that the adaptive immune system can indeed recognize tumor cells and destroy some of them. Secondly, the pressure imposed by the immune system can select tumor cell variants that successfully escape immune recognition. As a result, the immunological properties of tumor cells can undergo immunoediting (64).
This conclusion has both positive and negative implications. The immune system combats tumor cells; hence, the understanding of the mechanisms behind such targeting would allow an increase in its efficiency. On the other hand, in spite of the resistance of the perfectly healthy immune system, tumor cells can change their properties and escape immune surveillance by several mechanisms (MHC downregulation, decreased tumor antigen expression (65), or induction of anergic CTLs). As it became evident from these knockout models, the immune system is capable of targeting tumors in vivo, but it attempts to do so amidst a great deal of immunosuppressive and immune escape avenues that are available to genetically unstable and biologically heterogeneous tumor cells (66, 67).
1.5 Applications of Syngeneic Versus Human Xenograft Models
The development of clinical approaches to cancer immunotherapy has relied heavily on preclinical data gathered from experimental animal models. An ideal preclinical model for the evaluation of immunotherapy would need to exhibit predictable and reproducible intracranial growth patterns, mirror the histopathological and biochemical aspects of human gliomas, and exhibit minimal endogenous antitumor immunity in tumor-bearing hosts. The implantation of rodent glioma cells has proven to be an excellent intracranial glioma model due to efficient tumorigenesis and reproducibly accelerated growth rates (68). Syngeneic murine models (i.e. GL26 cells in C57BL6 mice (69, 70), SMA-560 cells in VMDK mice (71), CNS-1 cells in Lewis rats (69, 72), and F98 and RG-2 cells in Fisher rats (73–75)) are all minimally immunogenic (do not elicit a response capable of rejecting tumors spontaneously in implanted hosts) and constitute excellent tools for studying immunotherapeutic responses of gliomas (69, 75–77). Furthermore, because these animal models have an intact immune system, they are a valuable tool to test cross-reactivity of immunotherapeutic approaches with normal antigens and potential autoimmune or inflammatory toxicities associated with treatment (76–78). As an extension to syngeneic models for immunotherapeutic evaluation, spontaneous genetically engineered mouse (GEM) glioma models (79, 80), based on loss or increased expression of relevant genes implicated in human glioma pathogenesis, provide a unique opportunity to examine the pathologic and molecular alterations associated with tumor development and progression, while still preserving the function of the host’s immune system.
Human glioma xenografts implanted into immunocompromised mice have also been extensively employed in preclinical glioma research. Human xenograft models exhibit histopathological features that resemble human gliomas and retain the relevant gene amplifications (81). Also, these models are very useful for initial evaluation of novel imaging techniques (82) as well as new therapies for gliomas, including anti-angiogenic therapy (83, 84), chemotherapy (85), radiotherapy (86), targeted toxins (87), or oncolytic viruses (88–90). Although their xenogeneic nature impairs the study of immune-mediated antitumor strategies (due to the necessity of using an immunodeficient mouse to successfully engraft human tumor tissues), they do permit the assessment of the efficacy of therapeutic approaches in human glioma cells in an orthotopic animal model. The transfer of ex vivo manipulated lymphocytes has been used in a limited fashion for evaluation of immunotherapy in xenograft models and more recently, “humanized” mouse models consisting of immunodeficient mice reconstituted with human hematopoietic stem cells have been used to explore immunotherapeutic treatment of human cancers (91, 92).
1.6 Transgenic Mouse Models
Transgenic mouse models have allowed one to investigate the effects of different immunotherapy approaches on cancer. Human leukocyte antigen (HLA) transgenic mice can potentially overcome some of the main limitations inherent to human studies, such as the need for peripheral blood samples, the lack of controls, and the inability to control the influence of both MHC and non-MHC restricted genes on the immune responses. The HLA-A2 allele is one of the most common alleles in the general population (20–50% in Asian and Caucasian populations) and has been employed most frequently in transgenic mouse models (93, 94). In 1991 Vitelo et al. generated HLA A2.1/Kb transgenic mice (95), which have since been used by a number of groups to study CTL-mediated antitumor immunotherapy strategies (96). Because effector cells can recognize tumor cells and initiate their elimination, many transgenic strains have been activated to express modified human immune effector molecules. To study whether circulating naive CD4+ T cells could home to a tumor region and respond to idiotope (Id) peptides, Lauritzsen et al. established BALB/c mice transgenic for the αβ TCR specific for the λ2315 light-chain Id-peptide of a myeloma protein (97, 98). The transgenic CD4+ T cells displayed the characteristics of naive class II-restricted T cells in normal mice, proving that these mice were appropriate to study the role of naive T cells in immunosurveillance. By challenging the mice with different tumors, it was demonstrated that the transgenic mice were specifically protected against λ2315-expressing tumors.
Although B7-1 transgenic mice are usually generated to study the pathology of autoimmune diseases, the information provided by this model also holds implications in effective tumor immunotherapy. To explore the need for co-stimulation in autoimmunity, Allison et al. generated mice transgenic for B7-1 (99). Islets with IL-2 and confluent B7-1 expression were destroyed, whereas islets with IL-2 and patchy B7-1 expression showed selective killing on the B7-1+β cells. Islet-reactive T cells rejected syngeneic islet grafts, but only if these expressed B7-1. These results suggest that the eradication of a tumor might be dependent on continuously stimulated tumor-associated antigen (TAA)-specific CD8+ T cells locally by B7 molecules.
Preclinical findings from transgenic models have already found their ways to the patient, as they are currently being further evaluated in clinical settings. Transgenic lines expressing TAAs or oncogenes are extremely useful for the study of immunotherapeutic efficacy versus toxic effects. Furthermore, HLA and effector molecule transgenic mice permit the investigation of mechanisms involved in successful immunotherapy without the hindrance of tolerance. Crossing mice with both of these features will ultimately lead to the generation of animal models even more relevant to the treatment of human disease.
1.7 Oncolytic Viral Therapy Against Malignant Gliomas
The idea of “oncolytic viruses,” which are viruses used to target cancerous cells, stems from anecdotal reports of spontaneous cancer remissions that occurred coincidentally with natural infection or upon the use of live attenuated vaccines (100). Since then, an improved understanding of the molecular basis for viral host cell tropism, cytotoxicity, and cell type-specificity has opened up avenues for the targeted design of virally based anti-neoplastic strategies. Currently, the requirements for viruses in cancer therapy need to fall under at least one of the following domains: an inherently low human pathogenic potential (i.e. the orphan reovirus (101)), a veterinary pathogen with unknown human pathogenicity (i.e. vesicular stomatitis virus (102)), or a human pathogen genetically engineered to selectively kill cancerous cells without collateral cytotoxicity in normal cells (i.e. herpes simplex virus-1 (103)).
One experimental cancer therapy using the poliovirus receptor nectin-like molecule-5 (Necl-5) has been employed to determine viral tumor tropism and to initiate glioma cell killing. Treatment of glioma xenografts with intratumoral inoculation of PVS-RIPO (a prototype nonpathogenic recombinant poliovirus) in one study produced rampant tumor cell death, potent host-mediated inflammatory reactions against infected tumors, and rapid tumor decline (89, 104). These experiments documented two major components of oncolytic viral activity: direct viral tumor cell killing and resulting host inflammatory responses against the infected tumor.
Another preclinical study illustrated the potential usefulness of a replication-competent virus (reovirus) that killed glioma cells directly through cell lysis. The efficacy of this virus was demonstrated in established lines, in orthotopic (localized to where the cancer normally presents) and non-orthotopic glioma models, and in malignant glioma cell lines derived from surgical specimens. In contrast to SCID mice, no important toxic effects were found in nude mice, whose survival was dramatically prolonged after a single intracerebral administration of reovirus (105). The most important features of this potential anti-glioma treatment were its efficacy in an intracerebral location, its targeting of signaling pathways that are commonly activated in gliomas, and its ability to kill remote and invasive tumor cells.
Achieving success with oncolytic viruses in the clinical setting has been more challenging than the encouraging results achieved in many in vitro or animal studies. This translational difficulty stems from the fact that viruses depend on intricate relationships with their hosts that determine tropism for the intended tumor targets and the efficiency of targeted cell killing. Moreover, issues such as delivery, pre-existing immunity, or innate immune responses complicate therapeutic uses of viruses in patients (104).
1.8 Cellular-Based Immunotherapy Strategies in Animal Models
1.8.1 Active Immunotherapy: RNA-Transfected Dendritic Cells as Cancer Vaccines
Preclinical studies have shown DCs to be the most potent activators of de novo and recall responses in B and T cells and are thus regarded as one of the most promising entities for the realization of successful tumor immunotherapy (1–3). Our group has utilized the method of tumor-associated RNA transfection into DCs to prepare potent DC vaccines against MGs. A major advantage for using RNA-transfected DCs is the ability to extend this approach to a large number of patients despite limitations in obtaining tumor material for vaccine preparation. Building on existing technologies in molecular biology, we have shown that tumor mRNA can be isolated from a very small number of tumor cells, amplified, and used without loss of biological function (106). For instance, RNA isolated from tumor cells that were microdissected from pathology slides can be amplified and used to generate antitumor immune responses when transfected into DCs (107).
The efficacy of RNA transfection in eliciting immunity in vivo has been seen in several murine models, in which potent CTL responses developed in animals immunized with mRNA/lipid complexes (108–112). These responses were comparable to those achieved by infection with a live virus, indicating a physiologically significant level of immunity achieved by vaccination with RNA. In many studies, DCs transfected with RNA have proved superior to peptide-pulsed DCs in eliciting CTL responses against either peptide-pulsed experimental targets or intact tumor cells (4, 106, 107, 113). Furthermore, in tumor challenge experiments and stringent animal models of experimental metastasis, DCs transfected with in vitro transcribed RNA or ttRNA elicit protective antitumor immune responses (107, 112). Zhang et al. demonstrated the efficacy of tumor RNA-pulsed DCs in the 3LL lung carcinoma model and demonstrated that the vaccine’s antitumor effect could be enhanced by expressing the chemokine lymphotactin in the DCs (111). Despite the presence of known normal antigens within ttRNA preparations, autoimmune toxicity has not been observed in mice receiving tumor protective vaccinations of ttRNA-loaded DCs (114). RNA transfection offers a means to expand the scope of vaccination to other classes of tumors by providing an effective and readily measured method for generating tumor-specific T cell responses and concurrently reducing the amount of tumor tissue needed for antigen isolation by a significant magnitude. Because tumor mRNA-transfected DCs are potent stimulators of T-cell immunity in vitro and in vivo (106, 107, 112) and can function as targets in CTL cytotoxicity assays, they also offer a practical substitute for tumor cells in assays to test the immunological effects of specific antigens. Therefore, the use of DCs transfected with RNA for tumor immunotherapy is an effective vaccination platform in the preclinical and clinical setting, and we describe the methods to generate ttRNA-pulsed DCs and subsequently activate tumor-specific T cells in Sect. 2.
1.8.2 Adoptive Immunotherapy
Adoptive immunotherapy (AIT), which comprises the ex vivo expansion of effector cells and the return of tumor-specific T cells back into tumor-bearing hosts, has been shown in recent clinical trials to be a potent modality for achieving markedly high and sustained levels of tumor-specific T cells (115–117). The effectiveness of adoptive T cell therapy relies on the ability for adoptively transferred lymphocytes to localize to tumor sites and mediate the destruction of antigen expressing tumor cells (118–120).
Our preclinical data on adoptive immunotherapy against MGs with systemically administered lymphocytes support the notion that intracerebral trafficking and retention of T cells may be a limiting factor in the effectiveness of treating MGs by adoptive immunotherapy (27, 121, 122). To enhance intratumoral trafficking, we have explored the transfer of the chemokine receptor, CXCR2, into antigen-specific T cells to increase migration toward chemokines secreted in abundance by MGs (123–125) and CMV-infected cells (126) (e.g. IL-8, GRO-1-α, and the CMV viral chemokine, UL146). Tumor-specific T cell migration studies in SCID mice using fluorescently labeled human lymphocytes have revealed that limited entry into the CNS may be a potential barrier to AIT against MGs. However, we have shown that CXCR2 RNA-mediated gene transfer significantly enhanced T cell infiltration into the CNS in response to a variety of CXCR2-specific ligands in vitro and in vivo (55). These findings support novel methods for enhancing tumor-antigen-specific T cell trafficking into the CNS, which may be potent enough to counter the limitations of the CNS barrier as well as the tumor-induced state of immunosuppression.
1.9 Brain Tumor Stem Cells: A Future Immunotherapeutic Target
Malignant gliomas display incredible intratumoral heterogeneity, not only morphologically but also by grades of differentiation. Tumor heterogeneity may be derived from both genetic and nongenetic/epigenetic causes. Growing evidence from hematopoietic malignancies and solid tumors has supported the hypothesis that a subpopulation of cancer cells in each malignancy has greater potential for tumor initiation and repopulation (127–129). Although the nomenclature of these cells has been highly controversial due to the lack of definitive criteria, glioma stem cells (GSCs) have been described by several groups as the cancer stem cell population of MGs (129, 130). They are functionally defined by the capacity for self-renewal measured by serial neurosphere assays and tumor propagation by in vivo intracranial limiting dilution assays. GSCs have been implicated in several malignant behaviors. GSCs have been found to express elevated levels of vascular endothelial growth factor (VEGF) to promote tumor angiogenesis (131). This is of particular significance as bevacizumab (Avastin), a humanized neutralizing anti-VEGF antibody, has demonstrated activity against gliomas and was recently approved by the United States Food and Drug Administration for the treatment of recurrent or progressive gliomas (132–134). GSCs have also been characterized to be relatively resistant to radiation due to preferential activation of the DNA damage checkpoint and lesion repair (135), and other groups have described relative resistance of GSCs to chemotherapies (136, 137). These studies may explain in part how patients with a promising radiographic response universally suffer recurrence and/or progression of their cancers. Therefore, direct targeting of GSCs may improve the efficacy of conventional cytotoxic therapies as well as anti-angiogenic therapies.
Although GSCs appear resistant to radiation and chemotherapy, their susceptibility to immunotherapeutic attack has not been formally tested. A recent study in a murine glioma model revealed that the immunologic targeting of brain tumors using DCs pulsed with lysates from tumor cells grown in stem-cell culture media was more efficacious than DCs pulsed with tumor lysates from cells grown in standard culture, suggesting that the targeting of GSCs may be of therapeutic benefit (138). Thus, the identification of antigens expressed in GSCs and the development of immunotherapy that effectively targets these cells is a promising approach toward improving the outcome of immunotherapy for malignant gliomas.
1.10 Future Directions
Although in the past, the identification of tumor antigens has been difficult for many types of cancers, the data generated by techniques such as serial analysis of gene expression (i.e. from the Cancer Genome Anatomy Project) have revealed many new potential targets within public gene expression databases (139). These data require augmentation and verification, but, when additional tumor-specific antigens are identified, antigen-specific DC vaccine strategies may become applicable to many tumor types, with a multitude of specifically identified targets (14).
Immunologic targeting of tumor-specific gene mutations has the capacity to allow more precise eradication of neoplastic cells. Most well-characterized tumor antigens are overexpressed normal proteins that have triggered immunologic tolerance to some degree. This compromises their effectiveness as tumor rejection antigens and poses a risk of autoimmunity if these normal proteins are effectively targeted. Conversely, tumor-specific antigens derived from mutations in somatic genes are less influenced by central tolerance and less likely to be associated with autoimmunity. These mutations, however, usually arise randomly as a result of the genetic instability of tumors (140). As such, these mutations tend to be patient specific, and many are incidental to the oncogenic process. The epidermal growth factor receptor (EGFR) mutation EGFRvIII, however, is a consistent tumor-specific mutation frequently seen in glioma patients (141–143) and a broad array of other common cancers (144–146).
Although there is data to support the possible benefits of tumor-specific DC vaccination, there remain a number of issues that must be addressed to optimize this therapeutic modality. For example, in a study of 21 biopsy samples of human gliomas, only 47% of the tumors tested positive for EGFRvIII (147), and expression patterns even within these tumors are not homogeneous. This being true, vaccinations that target only one antigen may not target all tumors or all cells comprised in a tumor, and these vaccinations may subsequently select for the survival and proliferation of those cells that do not express the targeted antigen. Although the specificity of tumor-specific vaccination therapies may have the possible advantage of minimizing autoimmune complications, the heterogeneity of malignant brain tumors may limit the effectiveness of vaccinations targeting only one tumor-specific antigen. Furthermore, cross-presentation of nontargeted antigens could also lead to deleterious autoimmune responses. Conversely, multi-antigenic vaccines in this patient population have shown robust immunologic responses and encouraging clinical results without the induction of autoimmunity, and catastrophic autoimmune responses have not been reported to date (146).
The advantages of vaccinating with total tumor-derived material are that the identity of the tumor antigen(s) need not be known and that the presence of multiple tumor antigens reduces the risk of antigen-negative escape mutants. The potential benefit of using ttRNA is that it can be amplified from a small number of tumor cells. Furthermore, isolating bona fide tumor cells from patient specimens by ex vivo purification methods and combing this with the use of RNA subtractive hybridization techniques may reduce the concentration of self, potentially autoreactive antigens in the vaccine preparation (112).
Another outstanding limitation to the realization and efficacy of cell-based immunotherapies is the level of host immunosuppression imposed by the infiltrating tumor. Despite being restricted to the intracranial compartment, MGs elicit defects in host systemic cellular immune responses that are notably severe (148). These defects are characterized by dramatic reductions in both CD4+ T cell numbers and function. As a result, patients with MG suffer severe CD4+ lymphopenia (30, 149) with substantial T cell anergy characterizing the remaining CD4+ compartment (150). We have recently shown that a disproportionate presence of regulatory T cells (Tregs) amid CD4+ T cells provides an explanation for diminished T-cell function in these patients (30). Furthermore, our intracranial MG murine model recapitulates these findings. Specifically, tumor-bearing mice show dramatically reduced CD4+ counts in the peripheral blood, spleen, and cervical lymph nodes (CLNs), as well as increased Treg fractions and reduced T cell responsiveness (151).
Specific monoclonal antibodies (mAbs) that block immunosuppressive pathways in tumor-bearing hosts have demonstrated considerable benefit in preclinical and clinical immunotherapy settings. CTLA-4, a receptor constitutively expressed on Tregs and upregulated during activation of effector T cells, mediates downregulation of the immunologic response in activated lymphocytes (152, 153). We have shown CTLA-4 blockade to be a potent method for mediating the immunologic rejection of advanced gliomas in our murine models and reversing glioma-induced immunosuppression (154) and are currently pursuing evaluation of the clinical safety and efficacy of this modality in patients with MGs. In mice, CTLA-4 inhibition led to resistance to Treg-mediated suppression in effector cells without affecting the suppressive capacity of Tregs from CTLA-4 treated animals, indicating that despite constitutive expression of CTLA-4 on Tregs, the major impact of CTLA-4 blockade is on activated effector cells. Strategies to block the function of Tregs have shown promise in other clinical settings, and recent studies from our laboratory and others have demonstrated the potential benefits of Treg blockade using anti-CD25 mAbs in enhancing immunotherapeutic treatment of MGs (151, 155).
Given the presented strategies for immunotherapeutic intervention in preclinical models, a future focus for the eradication of MGs in patients would be to incorporate immunotherapeutic strategies in a combinatorial fashion with current standard of care modalities. In general, conventional therapies involve tumor resection, irradiation, and systemic chemotherapy (156). Unfortunately, most patients develop recurrence or progression after radiation treatment, and tumor radioresistance renders re-irradiation treatment less effective and potentially more toxic (157). Thus, cellular-based immunotherapies that can act in a synergistic fashion with radiation and chemotherapy sensitivity may improve outcomes in these patients.
The synergistic effects of vaccines with chemotherapy have been examined in clinical trials from major healthcare institutions (158, 159). In one study, clinical outcomes (survival and progression times) were analyzed retrospectively in vaccinated and non-vaccinated de novo glioma patients receiving chemotherapy. Patients receiving post-vaccine chemotherapy had longer survival times and exhibited significantly longer times to tumor recurrence after chemotherapy, relative to their own previous recurrence times, as well as relative to patients receiving a vaccine or chemotherapy alone (159). Based on the evidence that DC vaccination induces specific CTL targeting of drug-resistance-related TAAs and the aforementioned clinical observations, it is possible that therapeutic vaccination acts synergistically with subsequent chemotherapy to elicit tangible clinical benefits for glioma patients via the sensitization of tumor cells to therapeutic drugs after immune selection that has depleted former drug-resistant cells.
Immunization with cancer vaccines in particular offers advantages that other cancer therapy strategies do not. First, it is highly specific for cancer cells and, therefore, low toxicity should be expected. Second, it recognizes and eliminates cancer cells independent of their cell cycle phase. Third, tumors that developed drug resistance would still be a suitable target for immunotherapy. Fourth, vaccination offers the unique potential for a durable antitumor effect due to the phenomenon of immunological memory, potentially obviating the need for prolonged, repetitive cycles of therapy. Finally, immunotherapy offers the possibility of preventative immunization of high-risk patients (160). Given that synergistic effects of DC immunotherapy followed by chemotherapy have been observed in the clinical setting, further investigation of appropriate dosing schedules in preclinical models are necessary to examine the most potent, long-lasting, and safest regimen for targeting gliomas.
2 Methods Section: ttRNA-Pulsed DCs Activate Tumor-Specific T Cells in Animal Models
Dendritic cells are specialized APCs that play a pivotal role in the induction of T and B cell immunity (161). These cells have the exceptional ability to activate naive CD4+ and CD8+ T cells in vitro and in vivo. Because DCs require the presence of an antigen in order to initiate cytotoxic responses (162), when they are pulsed with tumor homogenate (161) or tumor-associated RNA (106), they are able to elicit a primary CTL response in vitro. Subsequent studies in vivo demonstrated that immunization of mice with DCs pulsed with an antigen can prime a CTL response that is tumor specific and provides protective immunity in treated mice. DC-based therapy is currently being evaluated in clinical trials for a variety of tumors (164).
Immunization using defined tumor antigens is, however, limited at present to a handful of human tumor types in which candidates for tumor rejection antigens have been identified. The advantages of vaccinating with total tumor-derived material are that the identity of the tumor antigen(s) need not be known and that the presence of multiple tumor antigens reduces the risk of antigen-negative escape mutants. Another benefit of using total tumor antigens in the form of mRNA is that it can be amplified from a small number of tumor cells (106, 107). Hence, DC vaccination may be extended to patients with MGs from which only a small (and possibly microscopic) biopsy can be taken for diagnosis. Furthermore, isolating tumor cells from patient specimens by ex vivo purification methods and combing this with the use of RNA subtractive hybridization techniques may reduce the concentration of self, potentially autoreactive antigens in the vaccine preparation. This would be of crucial importance for vaccinations with CNS tumor-derived antigens, as it may diminish the risk of severe autoimmune complications (112).
3 Materials
The materials used are listed below. Comparable products from other suppliers should also be effective.
3.1 Tumor Cell Lines and Animal Models
1.
SMA 560 cell line (spontaneous astrocytoma) and VM/Dk mice (71); 6–8 weeks of age (or syngeneic model of interest)
2.
Cell media: DMEM zinc option (ZO) medium (GIBCO BRL, Gaithersburg, MD) containing 10% (vol/vol) fetal calf serum (FCS), 25 mM HEPES, 2 mM l-glutamine, and 1 mM sodium pyruvate
3.2 DC Generation from Bone Marrow Cultures
1.
Syngeneic mice of desired number
2.
Sterile scissors, Mayo scissors, sterile chucks, sterile gauze
3.
Two pairs of sterile forceps
4.
70% Ethanol
5.
Halothane or Isoflurane and gas chamber
6.
50-ml Conical tubes (BD Falcon #352098)
7.
RPMI 1640 (Gibco #11875-085)
8.
Penicillin/Streptomycin (P/S) (Gibco) (store in 5.5-ml aliquots at −20°C)
9.
Heat-inactivated fetal calf serum (FCS) (Gibco)
10.
55 mM β-Mercaptoethanol (ME) (Invitrogen #21985-023)
11.
100 mM Sodium pyruvate (Gibco # 11360-070)
12.
10 mM Nonessential amino acids (NEAA) (Gibco # 11140-050)
13.
1 M HEPES buffer (Gibco # 15630080)
14.
200 mM l-Glutamine (Gibco # 25030081) (store in 4 ml aliquots at −20°C) (may be refrozen)
15.
GM-CSF (Immunex) (store at −20°C)
16.
IL-4 (Peprotech #214-14X) (store at −20°C)
17.
Cell lysis buffer (10×; Cell Signaling Technology #9803) diluted 1:10 in sterile H20
18.
100 mm Tissue culture dishes (BD Falcon #353003)
19.
70 μm Cell screens (BD Falcon #352350)
20.
25 G Needles (BD #305122) and 10 ml syringes (BD #309604)
21.
Trypan blue and hemocytometer
22.
6-Well tissue culture plates (BD Falcon #353046)
23.
Pipet-aid with 5-, 10-, and 25-ml pipets
24.
60-mm Tissue culture dishes (BD Falcon #353002)
25.
Opti-MEM media (Gibco # 11058-021)
26.
1.5-ml Eppendorfs (Brinkmann #220-36-320-4)
27.
2-mm Gene pulser cuvettes (BioRad #165-2086)
28.
Electroporator (BTX model ECM 830)
29.
Sterile disposable fine-tipped transfer pipets (Corning Samco #231-1S)
30.
FACS tubes with caps (BD Falcon #352054)
31.
Complete DC media (CDCM): To a 500-ml bottle of RPMI 1640, add the following:
27.5 (5%)mL FCS, 5.5 ml Pen/Strep (stored frozen), 2.0 ml l-glutamine (stored frozen), 550 μl β-ME, 5.5 ml sodium pyruvate, 5.5 ml NEAA, 5.5 ml HEPES. Thaw 1.1 × 106 U GM-CSF (should be stored in frozen aliquots) and 10 μg IL-4 (also stored frozen in aliquots). Pour entire bottle of media over a 0.2 μm, 500 ml sterile vacuum filtration unit (see Note 1).
3.3 Total Tumor RNA Extraction
1.
RNeasy kit (Qiagen, Valencia, CA)
2.
1.5-ml Eppendorf Safe-Lock tubes (for freezer storage of ttRNA)
3.4 Vaccination Using RNA-Pulsed DCs
1.
1× PBS (Gibco #10010-023)
2.
0.5-ml insulin syringes with needles (BD #305932)
3.5 CTL Priming and In Vitro Cytotoxicity Assay
1.
Target cells (tumor cells of interest) (5–10 × 106)
2.
Europium time-resolved fluorescence cytotoxicity assay kit
3.
Complete RPMI 1640 media (Gibco #11875)
3.6 Implantation of Brain Tumors
1.
Trypsin EDTA (0.25%)
2.
1× PBS (Gibco #10010-023)
3.
Methylcellulose
4.
250-μl Hamilton syringes (Hamilton, Reno, NV)
5.
Stereotactic frame (Kopf Instruments, Tujunga, CA)
6.
Bone wax and surgical staples
3.7 Statistical Analysis for Survival Data
1.
GraphPad Prism 5 (or a comparable statistical analysis program that can be used to generate survival curves)
4 Methods
4.1 Tumor Cell Lines and Animal Models
Using an appropriate tumor line and animal model, the effects of DC vaccines pulsed with total tumor RNA can be examined.
1.
A syngeneic spontaneous high-grade glioma model with the manipulation of murine DCs would be an ideal platform. For the purpose of these methods, we describe the immunotherapeutic effects of DC vaccines using the SMA 560 cell line, which was derived from an intracerebral transplant of a spontaneous astrocytoma from a VM/Dk mouse (H-2b) (71).
2.
Culture cell lines in ZO medium containing 5% (vol/vol) FCS.
3.
For murine DC extraction, use 6–8-week-old female mice, and maintain mice in a virus-free environment in accordance with the Laboratory Animal Resources Commission standards.
4.2 DC Generation from Bone Marrow Cultures
The procedure used in these studies was the same as previously described (110).
Day 0
1.
Sacrifice mouse in gas chamber. Perform cervical displacement on mice. Wash mouse by submersion in 70% ethanol.
2.
Place mouse on sterile gauze on sterile chuck. Cut the Achilles tendon on one hind leg. Then, beginning at this site, use scissors to extend skin opening up the entire rear length of the leg. Peel the skin from the ankle up the entire length of the leg, exposing the musculature. Cut the patellar tendon, found on the anterior surface. Turning the leg over again, bend the leg backwards at the knee, exposing the proximal tibia. Continue to push, exposing the tibia to the ankle. Holding the foot and tibia firmly in opposing hands, loosen the joint by gentle twisting until the tibia can be pulled in one swift motion from the foot. Clean the bone with gauze and place on ice in conical containing cold RPMI-P/S. Returning to the same leg, cut the remaining foot and lower leg muscles off at the knee, leaving only the upper leg. Slide the scissors in between the muscle and anterior surface of the femur. Spread the scissors to bluntly dissect the muscle off the bone. Repeat for the posterior surface of the bone. This will leave the distal femur and attached patella exposed. Grasp the exposed end of the femur and pull outwards from the body at a 45° angle to dislocate the hip. At this point, the scissors can be used to feel between the dislocated head of the femur and pelvis and to cut the femur away. Once the bone is removed, the patella may be grasped with the finger and folded back over the femur to remove it. Much of the musculature will come with the patella, and the femur may then be easily cleaned with gauze and placed in RPMI-Penicillin/Streptomycin (P/S). Repeat this procedure for each leg of each mouse.
3.
Once both legs have been removed, the sternum may be removed. Make a horizontal incision in the skin, over the area of the xiphoid process. The proximal flap may then be grasped and pulled rostrally, exposing the rib cage and shoulders. Pectoral muscles should be detached from the chest wall. Then, make a horizontal incision in the peritoneum, over the xiphoid process. Sliding the scissors rostrally under the sternum, bluntly dissect the organs away from the sternum. Scissors may then be used to cut along each side of the sternum, towards the head. Cut horizontally proximal to the manubrium to detach the sternum. Place it on ice in the same conical tube containing the long bones in RPMI-P/S. [Repeat steps 1 through 4 for each mouse].
4.
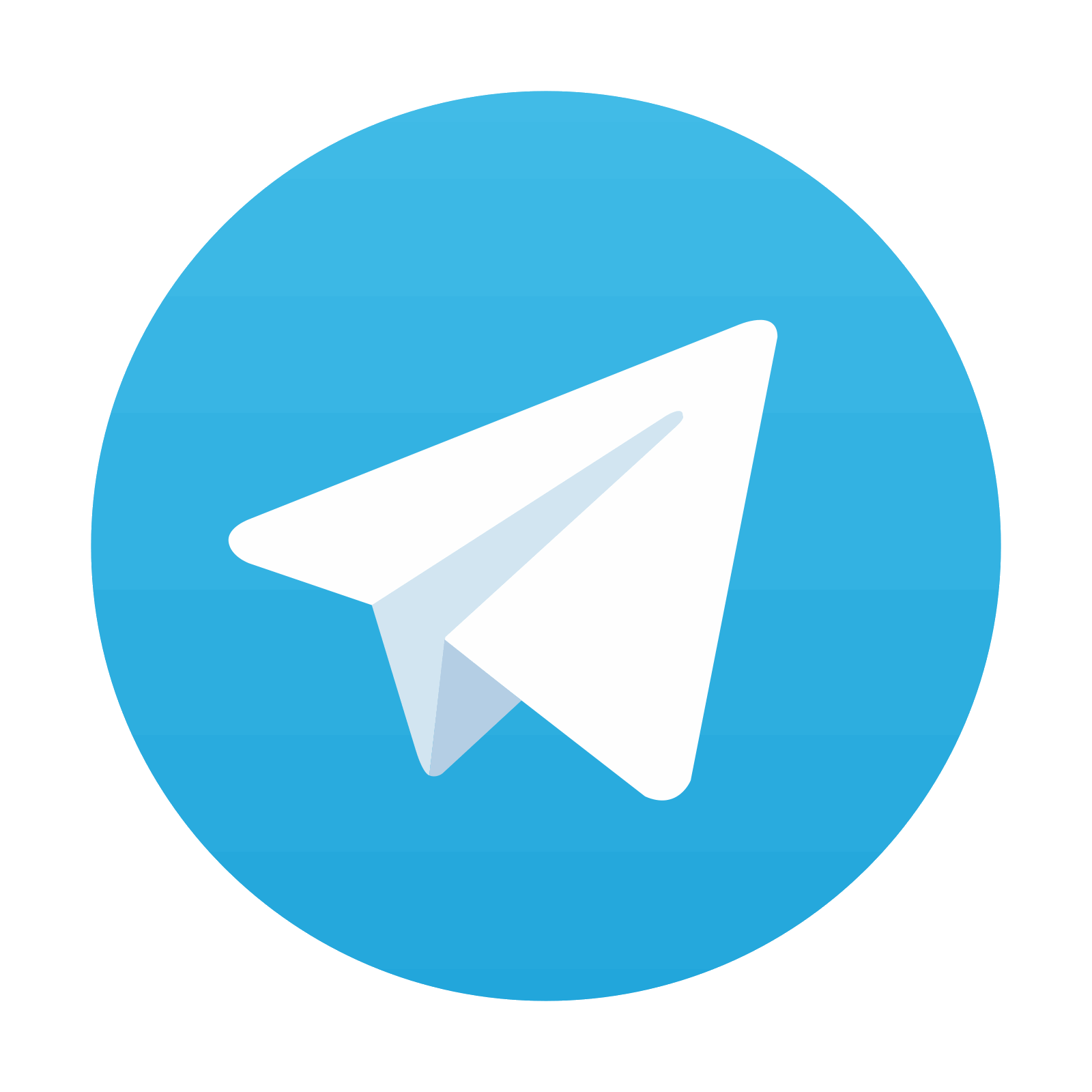
Following bone harvest, transport bones to tissue culture hood. Decant RPMI-P/S off the two conicals containing long bones and sternums. Replace with 70% ethanol, recap, and shake vigorously for at least one minto wash. Decant off ethanol and replace with cold RPMI-P/S. Shake again for 1 min.
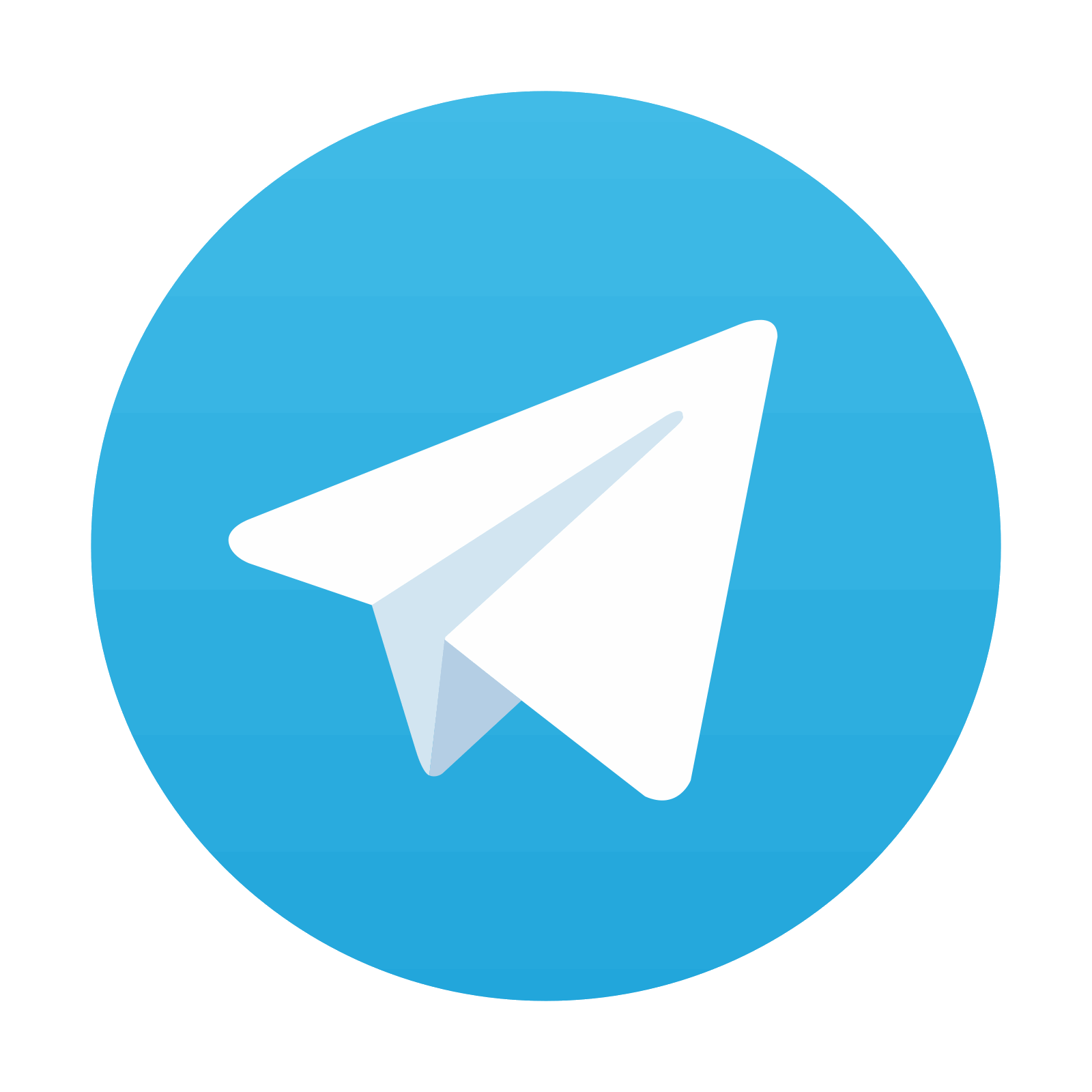
Stay updated, free articles. Join our Telegram channel
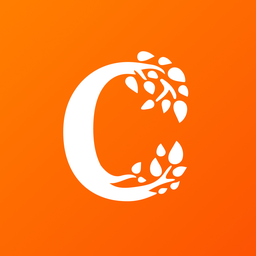
Full access? Get Clinical Tree
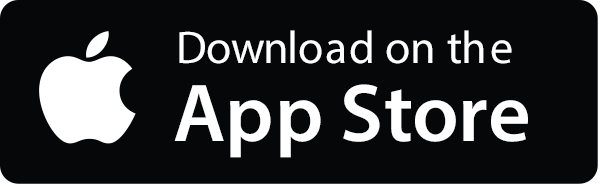
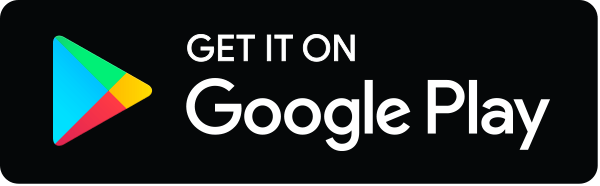
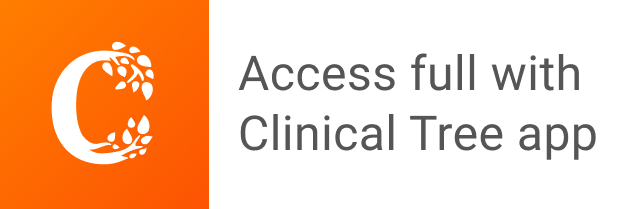