Fig. 1.
Schematic drawing of PET image signal generation. An intravenously injected imaging or reporter probe, such as a radiolabelled PET tracer, can be an enzyme substrate, a receptor ligand, an antibody, or a transporter molecule, which is retained at the target organ through (1) enzymatic modification followed by mechanical or chemical entrapment; (2) binding to a receptor or antigen; (3) cell uptake by a membrane transporter; or a combination of the above. The enzymes, receptors, antigens, or transporters can be expressed by endogenous genes or can be the product of an exogenous gene that has been introduced into the target tissue by a pretargeting vector.
These substrates or binding compounds, called PET (radio)tracers, probes or ligands, are labeled with PET decaying nuclides/positron emitters such as 11C, 13N, 15O, 18F, 64Cu or 124I. As such nuclei are commonly found in biological tissue, PET tracers can be chemically identical to or close analogues of naturally occurring molecules, making them especially suitable to interrogate in vivo biological processes.
A positron emitted from a PET probe travels a few millimeters in tissue before being annihilated by collision with a target electron, resulting in a pair of high-energy (511 keV) photons or gamma rays. The two gamma rays are emitted at nearly 180° from one another and travel in opposite directions throughout the subject before they are detected simultaneously by the PET detector(s) that are arranged in a ring-shaped array surrounding the subject. There exist two major principles for the technical design of PET detectors. The first one uses photon-sensitive scintillation crystals that convert the incoming gamma ray photons into light flashes and further into electrical signals by the photomultiplier tubes surrounding the scintillation crystals. The second principle is based on a multi-wire gas chamber in which the 511 keV photons are converted into electrons. These electrons are then multiplied within the gas by means of an electrical field (avalanche effect) and finally detected by anode wires. This detector principle is used in the quadHIDAC PET scanner (Oxford Positron Systems Ltd., Weston-on-the-Green, UK), which is used in our institution. A more detailed description of the technical aspects of this scanner can be found in (10). For both principles, the two photons that elicit signals at nearly the same time in detectors 180° apart from one another constitute a recordable event, defining a vector along which the positron-emitting probe lies. Multiple annihilations, detected as additional coincidence events, define additional intersecting vectors that identify the site of probe sequestration. These raw PET datasets are then corrected for signal attenuation due to absorption by tissue and finally used to mathematically reconstruct volumetric images of the positron-emitting probe (11).
Once PET images have been obtained, the PET data must be analyzed. The simplest method for data interpretation is qualitative imaging, which is mostly sufficient for tumor detection. However, qualitative data interpretation fails to give insight into in vivo tumor biology and to take full advantage of the inherently quantitative nature of PET data (12). The most practical method for PET image quantification is by calculating the standardized uptake value (SUV), defined as the ratio of tissue radioactivity uptake and the injected dose per unit subject weight. SUV describes the level of activity in a particular spot compared to activity elsewhere in the body. A SUV reading of one [1] is baseline or normal cellular activity. SUV is vulnerable to several major sources of variability, such as body composition and plasma glucose levels, length of uptake period and time of SUV evaluation, partial volume and spillover effect (13). Major reason for the continuous usage of SUV in preclinical research is that blood sampling is not necessary. The most detailed quantitative approach uses dynamic PET imaging and kinetic analysis. In this approach the blood clearance curve is obtained from dynamic images of a blood pool structure or from blood sampling and serves as the input into a compartmental model from which relevant kinetic parameters can be estimated (12, 14).
The most commonly used positron-emitting isotopes 11C, 13N, 15O and 18F have relatively short half-lives, in the order of minutes to hours, whereas the 64Cu and 124I have longer half-lives, from hours to days. Short-lived and long-lived isotopes have distinct advantages and disadvantages such as the necessity to have an on-site cyclotron and radiochemistry laboratory for tracer production in the case of short-lived isotopes.
Positron-emitting radionuclides are primarily used to tag small molecules that are recognized by enzymes or bind to cell receptors or membrane transporters. These enzymes, receptors or transporters can be endogenously expressed and this methodology is used in conventional PET imaging, e.g. imaging of cell metabolism with 2-deoxy-2-[18F]fluoro-d-glucose ([18F]FDG) targeted to the enzyme hexokinase or imaging of the activity of amino acid transporters with l-[S-methyl-11C]Methionine ([11C]MET) in subjects with brain tumors. These molecules can also be expressed by exogenously introduced genes (marker genes) and used for the evaluation of newly developed experimental therapies such as gene therapy protocols.
Advantages of PET imaging compared with other noninvasive imaging techniques are its (1) very high sensitivity (within the picomolar range), (2) excellent signal-to-background ratios, (3) quantitative and inherently tomographic nature with unlimited depth penetration, (4) availability of a broad range of applicable probes and (5) absence of toxicity due to the low absolute amount of substance used (15). However, nuclear imaging has (1) only moderate spatial resolution (currently in the submillimeter range for preclinical applications and of 2–4 mm in clinical practice) when compared to magnetic resonance (MR) imaging (1–10 μm for ultrahigh-field MR scanners), (2) demands sophisticated instrumentation with committed personnel, and usually can (3) only be performed at facilities that have an on-site or nearby cyclotron for delivery of radioisotopes and the required radiochemical laboratories for the preparation of radiotracers. Furthermore, the exposure of studied subjects to radiation leads to the need of dosimetry and may limit the number of examinations that can be performed (16). As a consequence, regardless of the very promising potential of PET imaging especially in the field of molecular-genetic imaging, the technique is still not widely available compared to other noninvasive imaging modalities such as CT, MRI or ultrasound.
3 PET Imaging of Tumor Biology
PET imaging has the unique ability to provide not only noninvasively and quantitatively but also longitudinally disease-specific information related to disease initiation, characterization, progression and response to therapy.
This information can be allocated to more general biochemical and physiological processes related with tumor growth (such as energy or membrane metabolism, protein or DNA synthesis, vascular function or hypoxia) or to processes related to disease-specific molecular-genetic alterations involved in apoptosis, angiogenesis and other signaling pathways.
As a result of oncogenic mutations tumor cells undergo “metabolic transformation” leading to enhanced bioenergetic and biosynthetic activities (17, 18). Due to their metabolic autonomy tumor cells are taking up nutrients (glucose and glutamine) and allocate them into metabolic pathways that convert them into biosynthetic precursors (amino acids, nucleotides and lipids) that support their growth and proliferation. Tumor cells can achieve this phenotype through changes in the expression of enzymes that determine metabolic flux rates, including nutrient transporters (e.g. GLUT1 glucose transporter, amino acid transporters) and enzymes (e.g. hexokinase, thymidine kinase 1, choline kinase).
[18F]FDG PET is a standard PET imaging modality used to measure local or regional glucose metabolism. [18F]FDG PET is based on the uptake principle of the [18F]FDG tracer, a direct analogue of glucose. As tumor cells rely on aerobic glycolysis for a major part of their energy consumption, [18F]FDG PET detects the increased energy demand associated with tumor growth. In neuro-oncology it has been shown that an increased intratumoral glucose consumption, as measured with [18F]FDG PET imaging, correlates with tumor grade, cell density, biological aggressiveness and patient survival, both in primary and recurrent gliomas and that [18F]FDG PET imaging can be used to detect the metabolic differences between normal brain tissue, low- and high-grade gliomas and radionecrosis (19). [18F]FDG PET can also be used to predict the outcome to chemo- and radiotherapy (20). However, due to the high background uptake of [18F]FDG in normal brain tissue as well as in inflammatory cells, the specificity of [18F]FDG PET is relatively low.
Due to the enhanced biosynthetic activity in tumor cells these cells display an increased protein, DNA and membrane synthesis. As a consequence, the demand for amino acids, nucleosides and lipids, the respective building blocks for proteins, DNA and membranes, is increased and radio-labeled analogues of these molecules have been used in the management of malignancies (e.g. for tumor detection, staging and restaging and therapy follow-up). Nearly all amino acids have been radio-labeled but only a few are used systematically for cancer research purposes. Among these, labeled methionine and tyrosine are the most commonly used due to their ease of production and limited number of in vivo metabolites (21, 22). Uptake of [11C]MET and [18F]fluoroethyltyrosine ([18F]FET) have been shown to correlate with in vitro cell proliferation, Ki-67 expression, nuclear antigen expression and microvessel density (23) and can be used for glioma grading (24), to differentiate recurrent tumor from radiation necrosis (25) and to evaluate therapy response and predict outcome (19, 26). Also a variety of nucleic acids have been radio-labeled as PET tracers to study DNA turn-over and thus reflect cellular proliferation (1, 27). Of these, 18F-labeled 3′-fluoro-3′-deoxythymidine (FLT) is the most intensively studied. FLT accumulates in proliferating tissues following phosphorylation to FLT-monophosphate (FLT-MP) by the S-phase specific thymidine kinase-1. It has been demonstrated in many types of cancer, including malignant glioma, that [18F]FLT uptake in vivo indeed is a measure of tumor proliferation activity as there exists a significant correlation between [18F]FLT uptake and the in vitro proliferation marker Ki-67 (28–34). Both [18F]FLT and [11C]MET are more selective in detecting brain tumors compared to [18F]FDG, which is nonspecifically retained in brain tissue, due to its high glucose metabolism. [18F]FLT and [11C]MET, on the contrary, reveal only a low uptake in healthy brain tissue (19). Furthermore, amino acid and nucleoside analogue tracers have been shown to be superior to [18F]FDG to differentiate between tumor and inflammation (35, 36), which is of high importance as the increased glucose metabolism of inflammatory tissue is the main source of false-positive [18F]FDG PET findings in oncology.
High cellular uptake of choline, an important precursor in the synthesis of cell membranes, is a common feature in tumors and reflects the increased cell proliferation associated with malignant transformation and radio-labeled choline variants have been used for PET imaging of cancer (37). Although choline PET imaging can be considered as a general tumor imaging modality, to date studies mostly focused on the evaluation of prostate cancer and more recently also of brain tumors. In malignant glioma, areas of increased uptake of 18F-labeled choline seem to correspond with areas of elevated choline metabolites as determined by MR spectroscopy (38) and are able to differentiate between recurrent tumor and radiation necrosis (39, 40).
Tumor angiogenesis and tumor hypoxia, both related with poor disease prognosis, are other key factors in tumorigenesis that are tightly correlated and that can be evaluated with PET imaging. Tumor-associated angiogenic processes result from the imbalance between pro- and antiangiogenic factors that are triggered by hypoxia or genetic anomalies such as expression of oncogenes or tumor suppressor gene mutations (41). Early PET imaging studies have used tumor perfusion and blood volume imaging with radio-labeled water or carbon monoxide as a measure of tumor vasculature and angiogenesis (12). More recently PET probes have been developed that can target directly the molecular events associated with angiogenesis, such as the vascular endothelial growth factor (VEGF)/VEGF receptor (VEGFR) signaling pathway, matrix metalloproteinases or integrins (42). Tumor hypoxia can be detected by the use of PET tracers sensitive to low tissue oxygen such as radio-labeled misonidazole derivatives (43) or by direct imaging of hypoxia-related molecular events such as the induction of hypoxia-inducible factor-1α (HIF-1α) (44).
Aberrant apoptosis is another integral component in cancer growth and is regulated by a complex proteolytic cascade triggered by cell surface death receptors (extrinsic pathway) or via mitochondria (intrinsic pathway). Both pathways eventually lead to activation of caspases (e.g. caspase-3) and to redistribution of phosphatidylserine to the outer cell membrane and represent processes that can be targeted by specific PET probes (45).
Recent advances in genomics, proteomics and metabolomics have revealed several other signaling pathways involved in tumorigenesis and much effort has been put in the development of imaging probes specifically directed to these pathways (46). One of the best studied is the epidermal growth factor (EGF)/epidermal growth factor receptor (EGFR) pathway and EGFR dysregulations have been identified in up to 60% of patients with glioblastoma and correlated with poor prognosis (47). Over the last decade several novel molecular therapies targeted to this signaling pathway have been developed and tested (e.g. monoclonal antibodies against EGFR) with contradictory results (48, 49). Moreover, a number of PET probes have been developed to directly detect the expression activity of EGFR and thus enable a specific imaging read-out for anti-EGFR therapy (50–52). For instance, Lee et al. radiolabeled a chimeric antibody (ch806) that selectively binds to the constitutively active EGFR mutant de2-7 EGFR (or EGFRvIII) and demonstrated that this radioligand (124I-IMP-R4-ch806) allows high-resolution imaging and quantification of de2-7 EGFR expression in xenograft gliomas (50). Precise evaluation of tumor-related molecules that can be targeted by specific drugs will not only allow evaluation and quantification of treatment efficacy, but will also allow efficient patient selection on the basis of the presence/absence of the drug target and guide optimal drug dosage and time course for treatment.
4 PET Imaging of Marker Gene Expression
The treatment of cancer using gene therapy approaches continues to attract interest of many researchers and promising results have also been reported for brain tumors in selected cases (53).
As the success of gene therapy protocols mainly depends on the specific and adequate delivery of therapeutic genes to target tissue and the local temporal and spatial control and regulation of the level of gene expression, the development of methods capable of imaging vector distribution and gene expression noninvasively, sensitively and longitudinally have received much attention over the last years (54).
Molecular biologists and cell biologists have, for many years, been using marker genes such as beta-galactosidase or green fluorescent protein (GFP) to analyze gene expression and regulation in cell cultures. With the development of dedicated small animal PET scanners, marker (aka reporter) genes have been developed whose expression can be monitored noninvasively in living animals.
Several genes have been proposed as potential marker genes for radiotracer-based molecular imaging (11, 55, 56). These marker genes can be broadly classified as genes whose gene product is an intracellular protein (e.g. enzymes) or is associated to the cell membrane (membrane receptors or transporters). The most widely used PET reporter gene encoded enzyme is the herpes simplex virus type 1 thymidine kinase enzyme, HSV-1-TK (57). HSV-1-TK can, like mammalian thymidine kinase, convert thymidine to its phosphorylated form. However, HSV-1-TK can also convert acycloguanosines (such as acyclovir, penciclovir and ganciclovir) and uracil derivatives (such as 2′-fluoro-2′deoxy-1-β-d-arabinofuranosyluracil—FIAU) to their phosphorylated derivatives, unlike the endogenous thymidine kinase. Once phosphorylated these derivatives are unable to exit the cells. Thus, diffusible positron-emitting acycloguanosines or uracil derivatives (such as [18F]-labeled 9-[4-fluoro-3-(hydroxymethyl)butyl]guanine, [18F]FHBG, or [18F]-labeled FIAU, resp.) are converted to trapped substrates by the HSV-1 thymidine kinase PET reporter gene product enabling the site-specific quantification of the HSV-1-TK enzyme activity and thus reflecting the level of reporter gene activity. The main advantage of the use of the herpes simplex virus type 1 thymidine kinase gene (HSV-1-tk) in gene therapy protocols is the fact that this gene can be simultaneously used as an imaging gene and as a therapeutic gene. The phosphorylated acycloguanosines, following conversion to their di- and tri-phosphate forms by HSV-1-TK, can kill cells either by blocking DNA synthesis or by causing chain termination. Most commonly ganciclovir is used as prodrug in this suicide gene therapy paradigm. However, at the trace levels utilized for PET reporter gene imaging, no physiological consequences of radiolabeled acycloguanosine administration are observable. Therefore, HSV-1-tk has been studied extensively for gene therapy purposes due to the fact that it can serve as a suicide gene and reporter gene simultaneously depending on the administered substrate (ganciclovir (GCV) and [18F]FHBG or [18F]FIAU) (58–60). The HSV-1-tk reporter gene has been used to track tumor cells, immune cells or stem cells, to assess tumor growth and to evaluate response to all kinds of therapies (cytotoxic, cytostatic, radiation therapy, chemotherapy, gene therapy) (54, 61) even in clinical application (58). Other PET reporter genes used for gene therapy purposes include the dopamine D2 receptor (D2R), the somatostatin type 2 receptor (SSTr2) and the sodium iodide transporter (NIS) genes (54). Especially the latter two have attracted much interest as these genes can also be used as therapeutic genes (radionuclide therapy) (62, 63).
However, in most imaging-directed gene therapy paradigms the marker gene does not have a therapeutic role itself but is coupled to an independent therapeutic gene, e.g. cytosine deaminase, interleukines or other cytokines. In such a vector system the expression of the marker gene is directly linked to the expression of the therapeutic gene. This means that direct localization and quantification of the marker gene reveals an indirect localization and quantification of the proportionally co-expressed therapeutic gene and allows the noninvasive determination of the tissue-dose of vector-mediated gene expression and, hence, the gene therapeutic capacity of the delivery system (60, 64, 65). The co-expression of 2 or more transgenes is generally achieved by using dual promoters, by inserting an internal ribosomal entry site or by fusing the transgenes into a single translational cassette within the gene construct or vector system.
One of the major disadvantages of reporter gene imaging is the fact that the reporter gene must be delivered (“pretargeted”) to the target tissue by the use of transfection or transduction (Fig. 1) and such manipulations may hamper their clinical applicability. A reporter gene construct usually includes transcriptional control elements that can initiate and regulate reporter gene expression. Two general groups of promoter–enhancer control elements are recognized: one group comprises “constitutive” promoters that result in a continuous expression of reporter genes, the other group comprises “inducible” or “tissue-specific” promoters that respond to specific endogenous transcription factors and transcription-regulating complexes (56). The former have typically been used to monitor gene delivery and expression. The latter are generally used to monitor changes in cellular or physiological processes in tumors, which affect the activity of the inducible promoter and thus the concentration of an imaging-detectable marker. Gene reporter imaging can thus be made cell or tissue specific, providing a valuable insight into the specific molecular/genetic machinery of tumors (16). Moreover, the use of tissue-specific promoters within the vector system allows for a more refined tissue transduction, reducing side-effects to neighboring nontumor cells.
5 Some Applications of PET Imaging in Preclinical Brain Tumor Models
Noninvasive, repetitive, and quantitative imaging of biological properties has been employed in the clinic for cancer detection, staging, and management for many years using structural and functional imaging techniques such as CT, MRI, ultrasound, SPECT and PET. However, noninvasive imaging techniques have not been employed very extensively in the study of small animal models of cancer (11). The recent development of dedicated instrumentation (μPET tomographs) and improved reporter genes for noninvasive imaging have paved the way for new paradigms to study cancer in small animal models with the potential for extrapolation to the clinic (translational research). Moreover, dedicated small-animal instrumentation allows well-proven techniques in clinical routine to be refined in preclinical research (reverse translation). For instance, for accurate and specific estimation of cell proliferation, a parameter that is necessary to monitor and characterize brain malignancies and evaluate treatment responses, dynamic imaging and kinetic analysis methods are necessary. Only by applying compartmental analysis to estimate tracer rate constants can the exact contribution of tracer delivery (dependent on tissue perfusion and blood–brain barrier permeability) and tracer metabolic retention (real indicator of tumor proliferation status) to the total tracer uptake be measured (23, 34). Such dynamic PET imaging is widely applied in clinical studies and could recently also be achieved in a preclinical glioma model. Bradbury et al. demonstrated the feasibility of a 3-compartment, 4-parameter model to characterize [18F]FLT kinetics in vivo in a genetically engineered mouse model of high-grade gliomas (66). The authors developed and implemented a compartmental modeling approach where tracer rate constants were estimated by use of image-derived input (left ventricular blood clearance) and tissue time-activity data (tumor uptake) and that was able to clarify the extent to which tracer transport and metabolism contribute to overall tumor uptake. Such exact and noninvasive quantification of tumor proliferation will be of great value to evaluate tumor response of novel targeted therapies.
Traditionally response to anticancer therapy is evaluated by analyzing only morphologic changes (Response Evaluation Criteria in Solid Tumors—RECIST criteria) induced at the target lesion (67). Although this approach is feasible for the assessment of cytotoxic therapies, the value of anatomic criteria for the evaluation of the efficacy of novel signaling pathway inhibitors is rather poor as these therapies often don’t provoke changes in tumor volume. Especially here lays the value of PET imaging with its ability to evaluate therapy-induced changes in tumor metabolic activity, gene expression profile, cellularity and vascularity and to evaluate new targets to be exploited as imaging biomarkers in cancer (68). One such new target is the translocator protein (TSPO), also referred to as peripheral benzodiazepine receptor (PBR). The TSPO receptor is located at the outer mitochondrial membrane and is involved in steroidogenesis (cholesterol transport), oxidative processes, immunomodulation (inflammatory responses and microglial activation), cellular proliferation and apoptosis (TSPO ligands may be useful anticancer drugs by inducing cell-cycle arrest and apoptosis) (69). This protein has been extensively used as a biomarker of brain injury and inflammation (70). Recently an increased expression of TSPO in astrocytoma has been established and TSPO expression could be correlated to the grade of tumor malignancy and patient survival (71) opening up the feasibility of TSPO specific ligands to be used as imaging biomarkers for diagnosis and follow-up as well as for anticancer treatment due to their pro-apoptotic effect (72). Most TSPO-ligand PET studies have been performed with the gold standard ligand [11C]-(R)-PK 11195, which however has some important drawbacks such as low signal-to-noise ratio (≤2:1 tumor-to-normal tissue ratio), low blood–brain barrier permeability and relative high nondisplacable tracer binding both in tumor and in normal brain tissue (73). More selective TSPO tracers have been developed over the last years (74, 75) and Buck et al. characterized the high-affinity ligand [18F]-N-fluoroacetyl-N-(2,5-dimethoxybenzyl)-2-phenoxyaniline ([18F]PBR06), as a candidate probe for the quantitative assessment of TSPO expression in glioma (76). In C6 glioma-bearing rats, [18F]PBR06 was found to preferentially accumulate in the tumors with only modest accumulation in contralateral brain tissue, could easily be displaced by cold ligand (75% displacement) and [18F]PBR06 uptake correlated well with TSPO protein levels as assessed by Western blotting and quantitative immunohistochemistry in tumor and normal tissue. Another target that has gained increased attention over the last years, especially since the advent of effective antiangiogenic drugs are integrins. Integrin αvβ3 is highly expressed on activated endothelial cells and solid tumor cells, not expressed on mature vessels or on nonneoplastic epithelium and expression is highly related to tumor angiogenesis and metastasis (77). Furthermore, αvβ3 integrins are a well-known therapeutic target for blocking tumor angiogenesis (78, 79) and αvβ3-targeted radiolabeled ligands thus have the potential to become highly valuable imaging biomarkers for the evaluation of treatment response. Many radiolabeled arginine–glycine–aspartic acid sequence (RGD)-containing peptides have been developed, which show a good correlation between tracer uptake and in vivo αvβ3 integrin expression (15). Radio-labeled RGD-peptides have been used to longitudinally follow brain tumor growth and associated angiogenesis (80) and to evaluate the effect of antiangiogenic therapy (81). Battle et al. demonstrated that an [18F]-labeled small peptide containing the αvβ3 integrin-binding RGD sequence can detect changes in human glioblastoma xenograft uptake after treatment with the antiangiogenic agent sunitinib as early as 2 weeks after treatment initiation and that this treatment effect can be detected significantly earlier than a concomitant reduction in tumor size (81).
Although imaging paradigms that directly monitor drug targets exploit the full potential of PET imaging, most imaging studies just monitor the metabolic or proliferative effect of molecular pathway inhibitory drugs or other experimental treatment therapies such as gene- or cell-based therapy. For instance, Veeravagu et al. evaluated the efficacy of a novel αvβ3 integrin-targeted radioimmunotherapeutic agent ([90Y]Abegrin) in murine xenograft glioblastoma models by imaging the effect on tumor metabolic activity ([18F]FDG) and cell proliferation ([18F]FLT) (82) and Assadian et al. performed [18F]FDG imaging to predict the therapeutic efficacy of three drugs with a distinct mode of action, alone or in combination (83). In the former study, [18F]FDG uptake was significantly reduced in animals bearing orthotopic U87MG tumors and treated with [90Y]Abegrin or unlabeled Abegrin, whereas [18F]FLT uptake was only reduced in the [90Y]Abegrin treated group. Animals receiving saline or [90Y]IgG treatment or animals bearing tumors with low αvβ3 integrin expression (HT29 colorectal carcinoma cells) did not show any difference in [18F]FLT or [18F]FDG uptake, indicating the specificity of the treatment. In the latter study that tested an HIF-1α inhibitor (YC-1), a proapoptotic agent (an activator of calcium-activated potassium channels—NS1619) and an alkylating agent (temozolomide), the authors showed that the therapeutic efficacy of YC-1 can be determined as early as 3 days after therapy by means of [18F]FDG PET imaging. Recently, the synergistic effect of paclitaxel, a nonspecific antimicrotuble agent, and tumor necrosis factor-related apoptosis inducing ligand (TRAIL) on glioblastoma tumor xenografts could be monitored with [18F]FDG PET imaging (84). The PET scans performed 5–6 days post therapy revealed continued tumor metabolic activity above background in mock-treated control tumors, and tumors exposed to paclitaxel or TRAIL monotherapy. However, tumors treated with combined paclitaxel and TRAIL showed no apparent increase in metabolic activity above background. Only in mice treated with combined paclitaxel and TRAIL a decrease in tumor volume could be identified on microCT images performed 3 weeks post therapy, in all other treatment arms tumor volumes increased.
Several gene- and cell-based therapies have been developed and tested for the treatment of brain tumors, even in clinical application (85–87). Although very promising in preclinical models, these treatment paradigms showed moderate and contradictory results in clinical application. Inefficient spatial or temporal tumor targeting, partial tumor coverage and/or tumor heterogeneity could have been reasons for these sparse results. Noninvasive imaging techniques, and especially PET imaging, have the ability to increase the efficiency of gene- and cell-based therapies by guiding all steps of such experimental protocols, from the guidance of vehicle application in relevant tumor areas, over biodistribution and delineation of the ideal therapeutic time window to response assessment of these experimental therapies (54) (Fig. 2a). PET imaging using tumor metabolism directed tracers (such as [18F]FDG, [11C]MET or [18F]FLT) can identify viable tumor tissue as the only meaningful target sites in case of direct application of viral vectors or cell vehicles (59, 60) and can monitor the therapy-induced changes in tumor metabolism. Furthermore, in treatment paradigms using reporter genes linked to suicide genes the exact localization, distribution and activity of the suicide genes can be evaluated by radiotracers specific for the used reporter gene, such as [18F]FHBG/[18F]FIAU in case of HSV-1-tk reporter genes or radiolabeled iodine or somatostatin analogues in case of NIS or SSTr2. More importantly, the level of reporter gene activity as measured by reporter probe uptake can be linked to the level of treatment response as measured by metabolic tracer uptake as early as 3 days after start of therapy (Fig. 2b) (60, 88). Thus PET imaging can monitor the expression level of therapeutic genes, which dependent on the efficacy of tumor transduction, and its correlation to the induced therapy response. The clinical applicability and usefulness of such imaging protocols could already been shown in glioma patients (58, 89). One of the major advantages of using combined reporter and suicide genes in cell-based therapies is the possibility to identify cell-induced tumorigenic changes, such as terratoma formation, in an early phase (90), leaving the opportunity to use the suicide gene as a backup safety measure by effectively eliminating the introduced cells by prodrug treatment as could be impressively shown by Cao and coworkers (91).
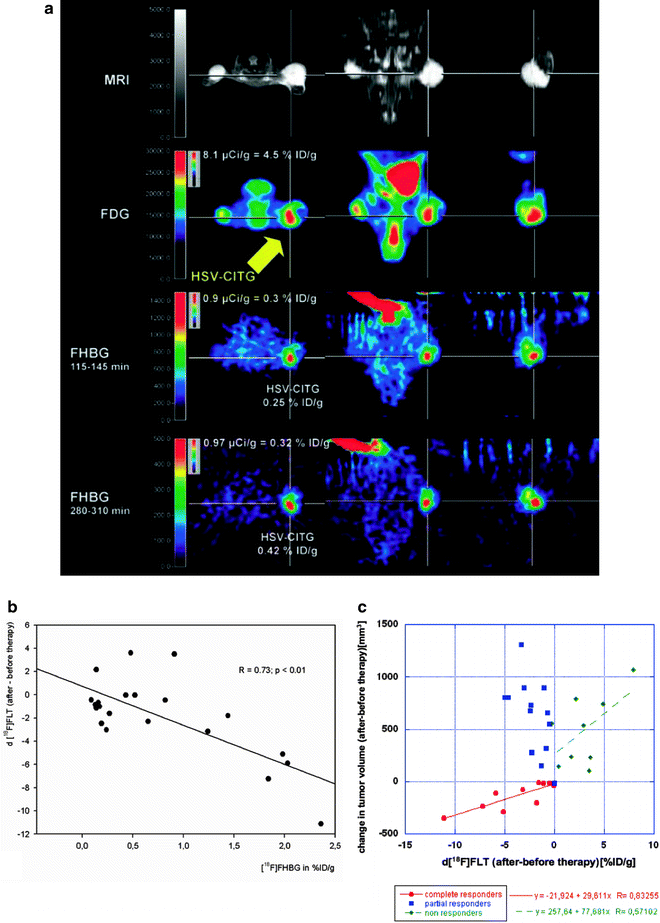
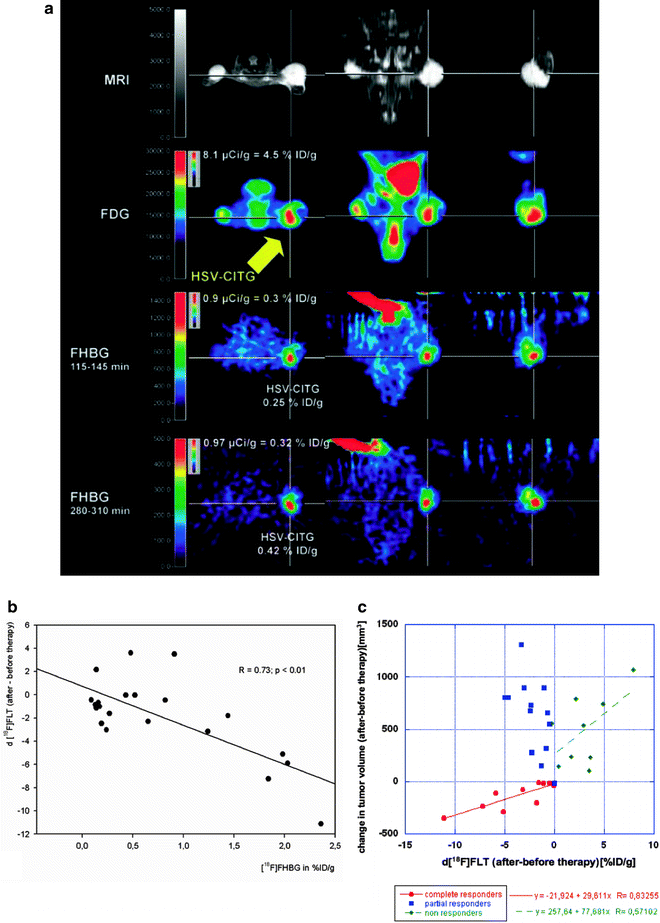
Fig. 2.
Imaging-guided gene therapy paradigm. (a) Experimental protocol for identification of viable target tissue and assessment of vector-mediated gene expression in vivo in a mouse model with three subcutaneous gliomas. Row 1: localization of tumors is displayed by MRI. Row 2: the viable target tissue is displayed by [18F]FDG-PET; note the signs of necrosis in the lateral portion of the left-sided tumor (arrow). Rows 3 + 4: following vector-application into the medial viable portion of the tumor (arrow) the tissue dose of vector-mediated gene expression is quantified by [18F]FHBG-PET. Row 3 shows an image acquired early after tracer injection, which is used for co-registration; row 4 displays a late image with specific tracer accumulation in the tumor that is used for quantification (adapted from Jacobs, Rueger et al., with permission (60)). (b) Response to gene therapy correlates to therapeutic gene expression. The intensity of cdIREStk39gfp expression, which is equivalent to transduction efficiency and tissue-dose of vector mediated therapeutic gene expression, is measured by [18F]FHBG PET (in %ID/g), and the induced therapeutic effect is measured by [18F]FLT PET (R = 0.73, p < 0.01). Therapeutic effect ([18F]FLT) was calculated as the difference between [18F]FLT accumulation after and before therapy (adapted from Jacobs, Rueger et al., with permission (60). (c) Relation between changes in volumetry and FLT uptake. There is a strong correlation between volumetry and change in FLT uptake (R = 0.83) for those tumors responding to therapy (complete responders) and a weak correlation (R = 0.57) for those tumors not responding to therapy (nonresponders) (adapted from Jacobs, Rueger et al., with permission (60).
6 Suggested Protocol for Small-Animal μPET Imaging of Energy Metabolism ([18F]-FDG), Proliferation ([18F]-FLT), and Amino Acid Uptake ([11C]MET)
6.1 Radiotracer
For research institutions without an own cyclotron, most [18F]labeled or other long-lived radiotracers can be either purchased from PET facilities with on-site production of radiotracers or be manufactured after purchase of the radio-labeled isotope, e.g. [18F]fluoride, from commercial vendors.
For radiotracers with short-lived isotopes, such as 11C or 15O, an on-site cyclotron and radiochemistry laboratory for ad hoc production of the required radiotracers are mandatory. At our institution no carrier added [18F]fluoride is produced via proton bombardment of [18O]H2O (nuclear reaction 18O(p, n)18F) in the cyclotron. After [18F]fluoride production and separation from [18O]H2O a KryptofixTM 2.2.2 solution (K222), that activates the [18F]fluoride anion for nucleophilic reactions in organic solvents, is added. For the radiosynthesis of [18F]FDG the precursor 1,3,4,6-tetra-O-acetyl-2-O-trifluoromethane-sulfonyl-β-d-mannopyranose reacts by nucleophilic substitution (SN2) with the activated [18F]fluoride yielding the target compound after hydrolytic (basic (92) or acidic (93)) deprotection of the acetyl groups. After purification via several cartridges [18F]FDG is diluted in a physiological buffer ready for injection. Similarly, one of the most effective [18F]FLT radiosynthesis starts with a SN2 reaction of an appropriate precursor (e.g. (5′-O-(4,4′-dimethoxytriphenylmethyl)-2′-deoxy-3′-O-nosyl-β-d–threo-pentofuranosyl)-3-N-Boc-thymine) and [18F]fluoride. After radiofluorination the intermediate is deprotected under acidic conditions and the resulting solution containing [18F]FLT is purified by semipreparative HPLC to yield [18F]FLT for injection (94).
A common method for the preparation of [11C]MET is based on the S-methylation of l-homocysteine with the reactive methylating agent [11C]iodomethane. Here, cyclotron-derived [11C]carbon dioxide (nuclear reaction 14N(p, α)11C) is transformed in a two step procedure to the radiosynthon [11C]iodomethane that can transfer his methyl-group to l-homocysteine even under mild reaction conditions using potassium fluoride on aluminum oxide as base. Finally solid phase extraction based purification and formulation provides the [11C]MET solution for injection (95).
6.2 PET Scanner
For small animal PET imaging a dedicated μPET scanner or a high-resolution clinical scanner is recommended. At our institution imaging is performed on the quadHIDAC PET scanner. This device acquires data within a large field-of-view of 16 cm (transaxial) and 28 cm (axial). Images are reconstructed into slices with voxel dimensions of 0.4 × 0.4 × 0.4 mm3. The spatial resolution of the quadHIDAC scanner is approximately 1 mm full width at half maximum (FWHM) in axial and transaxial direction using filtered backprojection image reconstruction, 0.7 mm FWHM (axial and transaxial) using one-pass listmode expectation maximization image reconstruction (96).
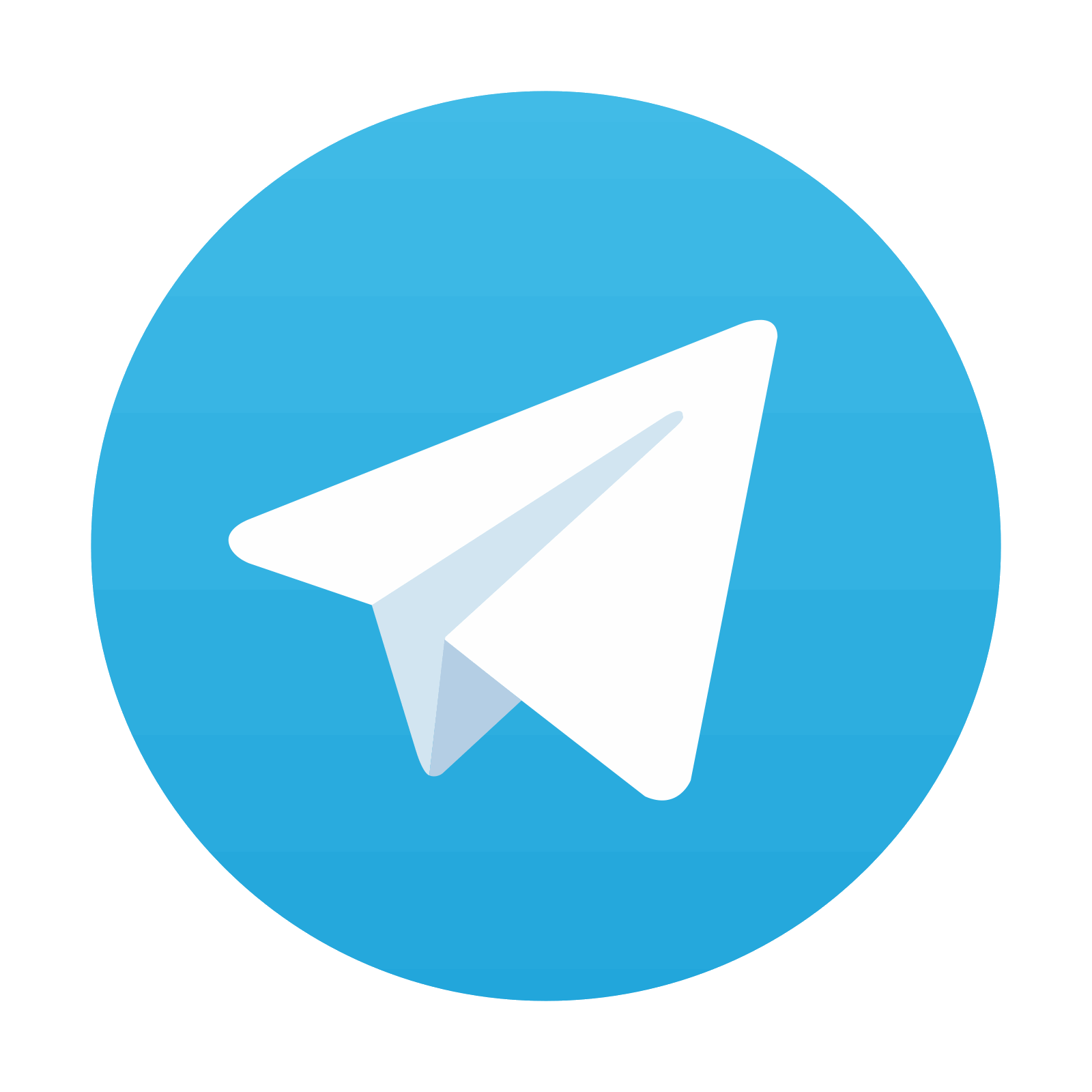
Stay updated, free articles. Join our Telegram channel
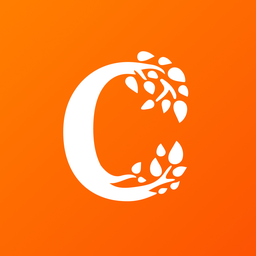
Full access? Get Clinical Tree
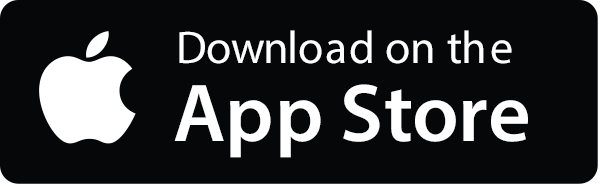
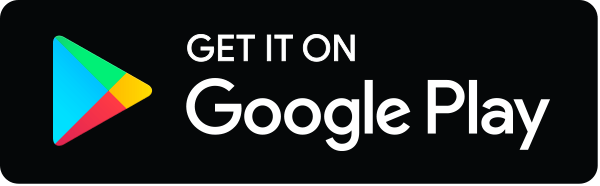