CHAPTER 14 The CNS consists of neurons, glia, ependyma, endothelial cells and pericytes of blood vessels, and the meninges (Fig. 14-1 and Box 14-1). Neurons vary in size, shape, and function, and their cell bodies are organized into functional groups such as nuclei, gray columns, and cerebral lamina. Neuronal processes called axons and dendrites traverse through the brain and spinal cord, the former often as organized bundles (tracts, fasciculi) forming synapses on cell bodies, dendrites, and axons of other functionally related neurons. It is estimated that there are 1 × 1011 neurons in the human brain. Each neuron makes approximately 10,000 synapses with other neurons; therefore there are about 1 × 1015 synapses in the human brain. The CNS is arranged to form two basic parts: the gray and white matter (Figs. 14-2 and 14-3). In the CNS, gray matter is found in the cerebral cortex, in the cerebellar cortex and cerebellar roof nuclei, around the base of the cerebral hemispheres (basal nuclei [often called basal ganglia]: caudate nucleus, lentiform nucleus [putamen, globus pallidus], amygdaloid nucleus, claustrum), and throughout the brainstem, often in nuclei. The gray matter is typified by numerous neuronal cell bodies, plus a feltwork of intermingled thinly myelinated axons and dendrites, their synaptic junctions, and processes of oligodendroglia, astrocytes, and microglia. This network of processes and synapses in the gray matter is referred to as the neuropil. The white matter consists of well-myelinated axons that arise from neuronal cell bodies in the gray matter and terminate distally in synapses or myoneural junctions, plus oligodendroglia, astrocytes, and microglia. In the cerebral hemispheres, white matter is located centrally; whereas in the brainstem, white matter is intermingled with gray matter (nuclei). In the spinal cord, white matter is located peripherally surrounding the gray matter. Fig. 14-2 Organization of the brain, gray matter, and white matter. Fig. 14-3 Organization of the spinal cord, gray matter, and white matter. Neurons: The structure and basic cellular biology of neurons is similar to that of other cells (Fig. 14-4); however, there are, as discussed later, some notable differences. The neuron consists of three structural components: dendrites, a cell body, and a single axon. The length of the axon varies, depending on the function of the neuron. The length of axons of motor or sensory neurons can be 10,000 to 15,000 times the diameter of the neuronal cell body, which results in these axons being several meters in length. The axon terminates in synaptic processes or neuromuscular junctions. Fig. 14-4 Neuron structure. Neuronal cell bodies vary considerably in size and shape, from the large neurons of the lateral vestibular nucleus, Purkinje cell layer of the cerebellum, and the ventral gray matter of the spinal cord to the very small lymphocyte-like granule cells of the cerebellar cortex (Fig. 14-5). Neuronal nuclei tend to be vesicular to spherical in shape, tend to be usually centrally located, and often, particularly in large neurons, tend to contain a prominent central nucleolus. Neurons contain focal arrays of rough endoplasmic reticulum and polysomes, termed Nissl substance, that are responsible for the synthesis of proteins involved in many of the neuron’s vital cellular processes such as axonal transport. Nissl substance is present in all neurons, regardless of the size of the cell body, but tends to be more prominent in those cells with voluminous cytoplasm such as motor neurons. Fig. 14-5 Variations in neuronal morphology, cerebellum, granule cells, and Purkinje neurons, normal animal. Axonal Transport: In most cells of the body, proteins and other molecules are distributed throughout the cell by simple diffusion. In neurons, simple diffusion alone is inefficient because synapses are a considerable distance away from the cell body of the neuron. As a result, molecules cannot diffuse the length of the axon; they must be transported the length of the axon to the synapse. In addition, there are no systems in axons or synapses to catabolize molecules resulting from normal metabolic processes in these structures. Thus these molecules need to be returned to the cell body for processing. These processes are facilitated in the axon by retrograde (toward the cell body) and anterograde (toward the synapse) axonal transport systems. Web Fig. 14-1 Axonal transport systems. As a result of these structural differences between neurons and other cells, neurons have developed axonal transport systems to efficiently move molecules and cellular organelles from the cell body through the axon to the synapses and their degradation products back to the cell body (Web Fig. 14-1). Axons can be longer than a meter in length, especially in an animal such as a giraffe. Lower motor neurons, whose cell bodies lie in the ventral gray horn of the spinal cord, and lumbar dorsal root ganglia, whose axons extend to the distal limb and to the caudal medulla, have the longest axons in the body. The neuron expends considerable energy and materials to move biologic materials up and down the axon. Alterations in the function of these transport systems can lead to neuronal dysfunction. Membrane Potentials and Transmitter/Receptor Systems: A fundamental activity of neurons is to modulate and effectively transmit chemical and electric signals from one neuron to another via synapses in the CNS or from one neuron to a muscle cell via junctional complexes, myoneural junctions, or motor end-plates in the PNS. The process of nerve impulse conduction is made possible by the establishment and maintenance of an electric potential across the cell membrane of the neuron/axon. Web Fig. 14-2 Resting and action potentials. Action potentials are caused by the movement of sodium and potassium ions across the neuron cell body/axon cell membrane. With an initiating event, sodium channels are first to open, and large concentrations of sodium ions enter the intracellular microenvironment (Web Fig. 14-2). Because sodium ions are positively charged, the polarity becomes more positive (−70 mV to −50 mV) and the neuron/axon becomes depolarized. Potassium channels open later in the depolarization process, concurrently with the closing of sodium channels. Potassium ions leave the cell and enter the extracellular fluid. These events cause repolarization of the neuron/axon and a return to a resting potential (−70 mV) via the membrane Na+/K+-ATPase pump. Alterations in these ion channels have been correlated with epilepsy in humans and will likely be discovered in animals. The action potential is a flow of depolarization that travels down the axon to synapses at the distal axon. When the axon lacks myelin, the flow of depolarization down the axon is called continuous conduction. When the axon is myelinated, the speed of conduction is determined by the degree of myelination of the axon and is called saltatory conduction. The diameter of unmyelinated axons can range from 0.2 to 1 mm with action potential velocities ranging from 0.2 to 2 m/sec, whereas the diameter of myelinated axons can range from 2 to 20 mm with action potential velocities ranging from 12 to 120 m/sec. The greater the degree of myelination, the faster the speed of impulse conduction down the axon. In unmyelinated axons, action potentials are conducted at a relatively “slower” velocity by the process of ion exchange (continuous conduction). In myelinated axons, action potentials are conducted at a relatively “faster” velocity by a mechanism called saltatory conduction. In this process, action potentials move down the myelinated axon using cable properties, like electric current flow in insulated copper wires. This method is fast, efficient, and requires less energy than ion exchange. However, the action potential would decay if axons were myelinated continuously along their length and likely would not reach synapses at full strength or at all. This decay is caused by loss of current across the cell membrane and capacitance properties of the cell membrane as the action potential travels down the axon. To minimize the decay of action potentials, axons are myelinated in segments called internodes. A gap, called the node of Ranvier, is formed between consecutive internodes and measures between 0.2 and 2 mm in length. At this gap, the action potential is restored to full strength by ion exchange. The node of Ranvier is highly enriched in sodium channels, and these channels are essential for impulse propagation via rapid action potential current restoration. Disease processes that disrupt myelination of axons will interfere with saltatory conduction, slow the action potential, and result in clinical dysfunction of the nervous system (see Fig. 14-21). Astrocytes: The functions of astrocytes in the CNS are regulation, repair, and support, as depicted in Fig. 14-6. Mature astrocytes differentiate from pluripotential progenitor cells during the development of the CNS. Astrocytes are the most numerous cell type in the CNS and have traditionally been classified into two types based on morphology. Protoplasmic astrocytes are located primarily in gray matter, whereas fibrous astrocytes occur chiefly in white matter. Microscopically, astrocytes have relatively large vesicular nuclei, indistinct or inapparent nucleoli, and no discernible cytoplasm with routine hematoxylin and eosin (H&E) staining (Fig. 14-7). With suitable histochemical stains, metallic impregnation, or immunohistochemical staining for glial fibrillary acidic protein (GFAP [the major intermediate filament in astrocytes]), the cell body and the extensive arborization and interconnections of astrocytic processes can be demonstrated. Processes vary from short and brushlike to long branching processes in protoplasmic and fibrous astrocytes, respectively (Fig. 14-8). These morphologic features and their corresponding histochemical and immunohistochemical staining reactions serve as important criteria for the classification of tumors of astrocyte origin. Fig. 14-6 Functions of astrocytes. Fig. 14-7 Histologic features of glial cells, ventral gray horn, spinal cord, horse. Fig. 14-8 Astrocytic processes, brain, cerebral cortex, normal animal. Regulation of the microenvironment: The microenvironment of the CNS must be under strict control to maintain normal function. Astrocytes are involved in homeostasis of the CNS and regulate ionic and water balance, antioxidant concentrations, uptake and metabolism of neurotransmitters, and metabolism or sequestration of potential neurotoxins, including ammonia, heavy metals, and excitatory amino acid neurotransmitters such as glutamate and aspartate. Interactions between astrocytes, microglia, and neurons orchestrate immune reactions in the brain. In this regard, astrocytes can express major histocompatibility complex (MHC) class I and II antigens, a variety of cytokines and chemokines, and adhesion molecules that modulate inflammatory events in the CNS. Astrocytes also secrete growth factors and extracellular matrix molecules that play a role not only in development but also in repair of the CNS. Repair of injured nervous tissue: In the CNS, reparative processes that occur after injury, such as inflammation and necrosis, are chiefly the responsibility of astrocytes. In these reparative processes, astrocytes are analogous to fibroblasts in the rest of the body. Astrocytes do not synthesize collagen fibers, as do fibroblasts. Instead, repair is accomplished by astrocytic swelling and division, and abundant proliferation of astrocytic cell processes containing intermediate filaments composed of GFAP, a process called astrogliosis. As an example, neuronal necrosis occurs in some viral diseases of the CNS. When neurons die, the spaces left by the loss of the neuronal cell bodies are filled and such spaces (<1 mm in diameter) are filled by processes of astrocytes. Larger spaces that form after injury, such as an infarct, are often too large to be filled and therefore exist in the CNS as fluid-filled spaces (cysts) surrounded by a capsule of astrocytic processes. Astrocytes will also attempt to wall off abscesses, but they are not as effective as fibroblasts and the capsule can be incomplete or weak (Fig. 14-9). In the case of direct extension of bacteria from the meninges or meningeal blood vessels, which contain or are surrounded by fibroblasts, respectively, fibroblasts play a larger role in isolating the inflammatory process. Fig. 14-9 Astrocytic repair, bacterial abscess, brainstem, sheep. Structural support of the CNS: Structurally, astrocytic processes provide support for other cellular elements and ensheathe and insulate synapses. Astrocytes also provide guidance and support of neuronal migration during development; thus, tracts and fasciculi of axons with similar functions are arranged and structurally supported by astrocytic processes. Processes of astrocytes (foot processes) also terminate on blood vessels throughout the CNS, forming a component of the blood-brain barrier. Astrocytes influence the induction of tight junctions between endothelial cells that serve as the structural basis for the blood-brain barrier. A dense meshwork of astrocytic processes also forms the glia limitans beneath the pia mater and is variably prominent in subependymal areas. During CNS development, cells termed radial glia provide a scaffold and guidance for migrating neurons. When development is completed, radial glia mature into astrocytes. Oligodendroglia: There are two types of oligodendroglia: (1) interfascicular oligodendrocytes and (2) satellite oligodendrocytes (satellite cells). The function of interfascicular oligodendroglia is myelination of axons, whereas the function of satellite oligodendroglia is thought to be regulation of the perineuronal microenvironment. Oligodendroglia have been compared with neurons with regard to their total cell size in that their processes occupy much more space than the cell body. Neurons have very long axons, which account for their size; oligodendroglia have extensive myelin sheaths, which account for their size. In H&E stained sections, oligodendroglia are often confused with lymphocytes because of the similarity of the morphology of their nuclei and cytoplasmic volume. Interfascicular oligodendroglia and perineuronal satellite oligodendroglia are located primarily in white and gray matter of the CNS, respectively (Fig. 14-10); however, interfascicular oligodendroglia can also be found along axons that traverse through the gray matter. The mature, small oligodendrocyte has a spherical, hyperchromatic nucleus (see Figs. 14-7 and 14-10). As with astrocytes, the cell body and processes of this cell do not stain with conventional H&E staining methods and can only be demonstrated following special procedures that include metallic (silver) impregnation and immunohistochemical methods. Fig. 14-10 Responses of glial cells to injury in H&E stained CNS sections. Most interfascicular oligodendroglia (see Fig. 14-10) are aligned in rows parallel to myelinated axons and are responsible for the formation and maintenance of segments (internodes) of myelin sheaths. One oligodendroglial cell can form as many as 50 different internodes of myelin, each of which can be located on many different axons (Fig. 14-11). Altered function of oligodendroglial cells, as occurs in infectious canine distemper virus (CDV) infection, can cause primary demyelination of these segments, resulting in severe neurologic dysfunction. Oligodendroglia also influence maturation and maintenance of axons and inhibit regeneration of established myelinated axons. Fig. 14-11 CNS myelin. Perineuronal satellite oligodendroglia (see Fig. 14-10) are adjacent to neuronal cell bodies and are also located around blood vessels in the gray matter. They are thought by some investigators to regulate the perineuronal microenvironment and respond to perturbation by proliferation. When the perineuronal microenvironment is altered or neuron cells bodies are injured, perineuronal satellite oligodendroglia, in an attempt to regulate the environmental perturbation, hypertrophy and proliferate in a process referred to as satellitosis. Similarly, alterations in the microenvironment of gray and white matter away from areas surrounding neuron cell bodies results in hypertrophy of oligodendroglia (see Fig. 14-10). Finally, satellitosis can be quite prominent in normal CNS in various areas of the gray matter. Microglia: The basic functions of microglia are immunosurveillance, immunoregulation, and reparative (phagocytic) activities after neural cell injury and death. The origin of microglia in the CNS has been debated for years. The current consensus is that the cells originate from circulating monocytes (mesoderm-derived) that enter and populate the CNS during embryonic development and early postnatal life, analogous to the formation of the monocyte-macrophage system in other organs. After entry into the CNS, the cells become amoeboid microglia, phagocytosing dead cells and cellular debris during remodeling and maturation of the CNS. Amoeboid cells then enter a quiescent stage and transform into ramified microglia. Ramified microglia constitute up to 20% of the glial cells and are present throughout the mature CNS, serving as sentinels of brain injury. Ramified microglia, also called resting cells, are most numerous in perineuronal and perivascular areas and in interfascicular locations in white matter. Evidence of pinocytosis in ramified cells suggests some role in maintaining the neural microenvironment. The principal function of microglia is phagocytosis, the initiation of and participation in the innate and adaptive immune responses, and in degenerative and inflammatory diseases of the CNS. Microscopically, ramified microglia have small, hyperchromatic ovoid-, rod-, or comma-shaped nuclei and no appreciable cytoplasm with routine H&E staining, thus the term rod cell is sometimes used to describe them (see Fig. 14-7). With special labeling techniques or metallic impregnation, ramified cells have a few delicate branching processes. The small hyperchromatic nuclei and nuclear shape distinguish microglia from astrocytes and oligodendroglia. However, microglia are often difficult to identify in H&E stained sections without some expertise in neuropathology. Ependyma (Including Choroid Plexus Epithelial Cells): The basic functions of ependymal cells, which line the ventricular system, are to move cerebrospinal fluid (CSF) through the ventricular system via movement of their cilia and to regulate the flow of materials between the CNS and the CSF. The ependyma is a single-layered, cuboidal to columnar, epithelium that lines the ventricles and mesencephalic aqueduct of the brain, and central canal of the spinal cord (Fig. 14-12). This layer of cells is therefore situated between the CSF and nervous tissue. Ependymal cells have cilia that project into the CSF and beat in a coordinated manner in the direction of CSF flow. Other structures, referred to as circumventricular organs, which include the choroid plexuses, are covered by highly specialized ependymal cells. The surface of ependymal cells that form the choroid plexus have microvilli (microvillus border) and cilia that occur singly or more often in groups of three or more. The choroid plexus epithelial cells also have specialized tight junctions (zonulae occludentes) that are a functional part of the blood-CSF barrier. In contrast to the choroid plexus, junctions between the conventional ependymal cells include gap junctions (transmembrane proteins form a pore, allowing communication between adjacent cells) and zonulae and fasciae adherentes, which permit movement of materials, such as proteins from the CSF, into the extracellular space of the brain. This cellular lining, however, is not a static membrane in that it regulates several processes that involve interaction between the CSF and brain. The functions include regulation of fluid homeostasis between the ventricular cavities and the brain, secretion and absorption of CSF, endocytosis, phagocytosis, and metabolism of substances such as iron resulting from the lysis of erythrocytes after hemorrhage into the ventricular system. Finally, ependymal cells have the structural and enzymatic characteristics necessary for scavenging and detoxifying a wide variety of substances in the CSF. Fig. 14-12 Ependymal and choroid plexus epithelial cells. Choroid plexus epithelial cells are modified ependymal cells. The choroid plexus epithelium is a single-layered, cuboidal to columnar, epithelium with a microvillus border (see Fig. 14-12). CSF is secreted from the microvillus border. Choroid plexus epithelial cells, along with capillaries and the pia mater, form the choroid plexuses that project into the lateral, third, and fourth ventricles. The basic function of choroid plexuses is to produce the CSF that fills the ventricular system and the subarachnoid space. CSF has two important functions: (1) to act as a “shock absorber” to mitigate the effects of trauma to the brain and spinal cord and (2) to deliver nutrients to and remove wastes from the CNS. Meninges: The meninges, which enclose the CNS, consist of three layers: the dura mater (outermost layer [pachymeninges]), the arachnoid membrane (mater), and the pia mater (innermost layer) (Fig. 14-13). Together, the arachnoid membrane and pia mater are frequently referred to as the leptomeninges, pia-arachnoid layer, or pia-arachnoid. The arachnoid membrane and pia mater are held together by bands of fibrous tissue called arachnoid trabeculae. This arrangement forms a compartment called the subarachnoid space in which CSF flows and which also contains blood vessels and nerves. There is also limited evidence based on studies in humans with neuro–acquired immunodeficiency syndrome that the brain has a primitive lymphatic system. The leptomeninges form a protective covering for the CNS and provide an external envelope filled with CSF that provides additional protection. Fig. 14-13 Organization of the meninges. The dura mater, once referred to as the pachymeninx (thick meninges), is a strong and dense collagenous membrane (Fig. 14-14). In the cranium, the dura consists of two layers that are fused with each other. The outer layer serves as the periosteum of the cranial bone, except in the areas of the venous sinuses (surrounded by dura) and falx cerebri, which is the longitudinal layer that extends ventrally between the two cerebral hemispheres. At the level of the foramen magnum, the two layers become separated; the outer layer continues to function as the periosteum of the vertebral (spinal) canal, and the inner layer forms the free dural membrane that surrounds the spinal cord. The inner aspect of dura mater is lined by elongated, flattened mesothelial-like cells. Except in neonates, there is no epidural (extradural) space in the cranial vault as there is in the spinal cord. There can be a “potential” epidural or extradural space in mature animals from hemorrhage caused by trauma. Fig. 14-14 Layers of the meninges.
Nervous System*
Central Nervous System (CNS)
A, Transverse section at the level of the thalamus, dog. Gray matter (darker areas) of the cerebral cortex lies beneath the leptomeninges on the external surface of the brain, whereas in the thalamus there tends to be a mixture of gray and white matter. Major white matter areas (light areas) include corona radiata, centrum semiovale, and corpus callosum of the cerebrum, and internal capsule and optic tracts bordering the lateral and ventral surfaces of the thalamus, respectively. B, Gray matter consists primarily of the cell bodies of neurons (arrows) and a network of intermingled thinly myelinated axons, dendrites, and glial cell processes. This network is referred to as the neuropil (N). Other components include oligodendroglia (perineuronal satellite cells) (arrowheads), protoplasmic astrocytes, and microglia. H&E stain. C, White matter primarily consists of well-myelinated axons (arrows) plus oligodendroglia (arrowheads) and fibrous astrocytes. The clear spaces surrounding large axons are artifacts formed when the lipid components of myelin lamellae are dissolved away by solvents in the process of embedding tissue in paraffin for sectioning. H&E stain. (Courtesy Dr. J.F. Zachary, College of Veterinary Medicine, University of Illinois.)
A, White matter in the spinal cord is located peripherally and divided into dorsal, lateral, and ventral funiculi. As a general rule, dorsal funiculi (D) consist of ascending sensory axons, lateral funiculi (L) have a mixture of sensory and motor axons, and ventral funiculi consist of descending motor axons (V). DGH, Dorsal gray horn; VGH, ventral gray horn. Histologically, the right side is a mirror image of the left side. The areas labeled B and C and contained within the boxes correspond to the areas illustrated in B and C. B, Transverse section of spinal cord, ventral gray horn, horse. The cell bodies of large motor neurons (arrows) are those of lower motor neurons and their axons extend in peripheral nerves to myoneural junctions that innervate skeletal muscle. H&E stain. C, Transverse section of spinal cord, ventral funiculus, horse. Because most axons course up and down the length of the spinal cord, in a transverse section, axons (arrows) are cut in cross section. They are surrounded by myelin sheaths whose lipid components are dissolved out during the preparation of paraffin embedded sections, resulting in clear spaces that are an artifact. H&E stain. D, Efferent spinal nerve (longitudinal section shown here), transverse section of spinal cord, ventral funiculus, dog. Axons of lower motor neurons leave funiculi (F) and assemble as nerve rootlets (arrow) eventually forming peripheral nerves that innervate skeletal muscle. H&E stain. (Courtesy Dr. J.F. Zachary, College of Veterinary Medicine, University of Illinois.)
Cells of the CNS
A, Basic cell biology and structure of neurons are similar to other cells in the body. Additionally, neurons have dendritic arborizations and an axon, specializations for the initiation, propagation, and transmission of impulses that underlie the basic function of these cells. B, The cytoplasm of the neuronal cell body has blue (basophilic [H&E stain]) granular material (rough endoplasmic reticulum) called Nissl substance (arrows). Nissl substance synthesizes proteins, including precursor neurotransmitter proteins and the structural proteins (neurofilaments), active in maintaining the integrity (length and diameter) of the axon. H&E stain. (A modified from Kierszenbaum AL: Histology and cell biology, St Louis, 2002, Mosby. B courtesy Dr. J.F. Zachary, College of Veterinary Medicine, University of Illinois.)
The granule cell neurons of the cerebellar cortex (arrowheads) are very small lymphocyte-like cells that have relatively little demonstrable Nissl substance when compared with Purkinje neurons (arrows) and large motor neurons (depicted in Fig. 14-4, B). H&E stain. (Courtesy Dr. J.F. Zachary, College of Veterinary Medicine, University of Illinois.)
Neurotransmitter vesicles and neurofilament proteins, synthesized in the rough endoplasmic reticulum and packaged in the Golgi apparatus are transported through the length of the axon and to synapses by kinesin. Kinesin is a microtubule motor protein that uses chemical energy from adenosine triphosphate hydrolysis to generate mechanical force and thus bind to and move attached to microtubules. Used vesicles and effete neurofilament proteins are returned along a microtubule (recycled) to the neuron cell body by cytoplasmic dynein, another microtubule motor protein. These transport systems are used by some pathogens (rabies virus, Listeria monocytogenes) to enter and spread within the CNS. (Modified from Kierszenbaum AL: Histology and cell biology, St Louis, 2002, Mosby.)
Nerve impulse conduction is made possible by the establishment and maintenance of an electric potential across the cell membrane of the neuron/axon. Resting membrane potential is established and maintained by differences in concentrations of potassium ions inside and sodium outside of the cell membrane. Sodium and potassium ions will leak across the cell membrane, and therefore concentration gradients are maintained by a sodium-potassium pump in the cell membrane. When an event depolarizes the cell membrane to a threshold level of approximately −50 mV, an action potential will occur. A, An action potential is initiated by an event that opens sodium channels, and the action potential is propagated along the cell membrane by the sequential opening of voltage-gated sodium channels in adjacent sections of the membrane. B, The action potential is regenerated in adjacent sections of the cell membrane as additional sodium channels open. Depolarized segments repolarize as sodium channels close and potassium ions move out of the cell. AP, Action potential. (From Copstead LC, Banasik JL: Pathophysiology: biological and behavioral perspectives, ed 2, Philadelphia, 2000, Saunders.)
Astrocytes provide structural integrity and regulatory oversight, as depicted in this diagram. They: 1, monitor and regulate fluid and electrolyte balances within neurons and surrounding extracellular space; 2, form the glial limitans at the base of the pia mater; 3, interconnect with other astrocytes to provide a system to monitor and regulate fluid and electrolyte balances throughout the CNS; 4, possibly participate in the formation and functions of the blood-brain barrier; 5, participate in the support of axon tracts of functionally related neurons; 6, monitor for and remove excessive release of neurotransmitters in synapses; 7, protect and insulate nodes of Ranvier; and 8, participate in the cerebrospinal fluid–brain barrier. In addition, astrocytes are a reparative (healing) cell after CNS injury with loss of tissue because nervous tissue, per se, is devoid of fibroblasts. Fibroblasts exist in the pia mater and other meninges. Everywhere else, healing depends on the astrocyte, which responds by increased length, branching, and complexity of cellular processes (astrogliosis). The astrocyte has many functions in the nervous system; one of them is to act in healing to produce a scar in attempts to isolate cavities and abscesses. Fibroblasts may also contribute to the formation of a scar, if this cell type is present, as it is in the leptomeninges. (Courtesy Dr. J.F. Zachary, College of Veterinary Medicine, University of Illinois.)
A neuronal cell body and its processes are in the center of the illustration. To the inexperienced, identifying specific types of glial cells in H&E stained histologic sections can be challenging. Astrocytes (arrows) have larger vesicular nuclei (dispersed chromatin) and the cell membrane and cytoplasm are rarely seen in nondiseased conditions. Thus these nuclei just seem to “sit” in the midst of the neuropil. The majority of nuclei in the neuropil here are astrocytic. Oligodendroglial cells (arrowheads) have smaller and dense round nuclei (condensed chromatin) often surrounded by a clear zone indicative of cell cytoplasm and a cell membrane. Oligodendroglial cells in gray matter are called perineuronal satellite cells; those in white matter are called interfascicular oligodendrocytes. Microglial cells are difficult to identify in H&E stained sections of the CNS, but they often appear as “rod cells,” which have small, dense elongated nuclei (dashed arrow). The light pink homogeneous tissue distributed in large quantities between these cell types is the neuropil. V, Blood vessels. H&E stain. (Courtesy Dr. J.F. Zachary, College of Veterinary Medicine, University of Illinois.)
Processes of astrocytes arborize extensively throughout the CNS (structures stained purple). Note that some of the processes are on the outside of blood capillaries (end feet) (arrows). A, Cell body of astrocyte. Holzer’s stain. (Courtesy Dr. M.D. McGavin, College of Veterinary Medicine, University of Tennessee.)
The abscess has a central core of necrotic debris (D) surrounded by a layer of inflammatory cells (I) and a less dense pink-staining zone representing an attempt by astrocytes and fibroblasts to form a capsule (A). This capsule is formed by fibrous tissue on the ventral and right sides, those sides closest to the pia, which contains fibroblasts. A fibrous capsule is absent from the dorsal and left sides of the abscess, adjacent to brain parenchyma. Here, there is no population of resident fibroblasts and the capsule is formed by astrocytes and their processes, which are often delicate and do not form an effective capsule (A). H&E stain. (Courtesy Dr. J.F. Zachary, College of Veterinary Medicine, University of Illinois.)
A, White matter. In nondiseased states, oligodendroglia in white matter are often arranged linearly (interfascicular oligodendroglia) (arrow) and are responsible for the formation of myelin around axons. In gray matter (not shown; see Fig. 14-17), oligodendroglia are dispersed as individual cells around neuronal cell bodies as perineuronal satellite cells (Fig. 14-10, B). H&E stain. B, Gray matter. When neurons are injured or there exists some perturbation of the perineuronal microenvironment, a long-held belief was that oligodendroglia around neurons hypertrophy and proliferate in a process referred to as satellitosis. Currently, there is no uniform agreement that these cells respond to neuronal injury in this manner. Perineuronal satellite oligodendroglia (arrows) surround a small degenerate neuron with condensed chromatin and little cytoplasm. H&E stain. C, White matter. Astrocytes (arrows) and oligodendroglia (arrowheads) have a limited repertoire of responses to injury in the CNS. Astrocytic proliferation can occur but is very difficult to determine in sections stained with H&E. Here, astrocyte nuclei are somewhat enlarged and appear more numerous than expected. H&E stain. D, Gray matter. Astrocytes respond to injury in hyperammonemia, such as occurs with hepatic encephalopathy, by forming astrocytes with enlarged, markedly vesicular (“watery”), often elongated nuclei called Alzheimer’s type II astrocytes (arrows). This type of astrocyte may occur in pairs that are surrounded by a clear space indicative of cellular swelling. H&E stain. (A courtesy Dr. M.D. McGavin, College of Veterinary Medicine, University of Tennessee. B to D courtesy Dr. J.F. Zachary, College of Veterinary Medicine, University of Illinois.)
Oligodendroglia myelinate axons within the CNS (also see Fig. 14-1). A, As depicted in this illustration, each oligodendrocyte sends out numerous cytoplasmic processes that repetitively encircle (myelinate) the portion of an axon between two nodes of Ranvier (internode) on the same and several different axons. Direct or indirect injury to an oligodendrocyte can result in “demyelination” of those internodes myelinated by that oligodendrocyte. This injury will slow the rate of conduction of an action potential, and depending on the site of the lesion may lead to clinical signs of neural dysfunction (ataxia, proprioception deficits). B, CNS nerves, longitudinal section. Axons and their neurofilaments (brown stain) and myelin (red stain) are well demonstrated by this immunohistochemical stain for neurofilaments and myelin basic protein. (Courtesy Dr. J.F. Zachary, College of Veterinary Medicine, University of Illinois.)
A, Ependymal cells are ciliated (arrows) and assist with the flow of cerebrospinal fluid (CSF) through the ventricular system. H&E stain. B, Choroid plexus epithelial cells (arrows) secrete CSF from a brush-border (microvilli) on the luminal surface. The surface of the choroid plexus also has cilia that occur singly or more often in groups of three or more on a single cell. H&E stain. (Courtesy Dr. J.F. Zachary, College of Veterinary Medicine, University of Illinois.)
The meninges, from outside to inside, are the dura mater, arachnoid mater, and pia mater as illustrated in the diagram. The arachnoid mater and the pia mater form the leptomeninges. These two layers of the leptomeninges also enclose the subarachnoid space, which contains the arteries, veins, and nerves and is filled with cerebrospinal fluid. The pia mater is attached to the surface of the CNS. Astrocytes and their foot processes underlie the pia mater and form the glia limitans (inset 1) and surround the endothelial cells that form the blood-brain barrier. As arterioles penetrate the cortex to supply the tissue with blood, they carry the pia and glia limitans with them for 1 to 3 mm until the arteriole structurally becomes a capillary. At this transition site within the cortex, the capillary penetrates the pia and is surrounded by the glia limitans, and the end feet of the astrocytes become part of the blood-brain barrier (inset 2). Components of the blood-brain barrier are capillary endothelial cells, basement membrane, and astrocytic foot processes, but the barrier is formed structurally by tight junctions between endothelial cells and functionally by specialized transport systems in these cells. (Courtesy Dr. J.F. Zachary, College of Veterinary Medicine, University of Illinois.)
A, Brain, dog. The dura matter is a thick opaque layer. Here it covers the rostral (cranial) half of the brain and has been dissected away from the caudal half of the brain to expose the underlying leptomeninges. In old animals, the dura mater often fuses with the periosteum of the calvarium, and at necropsy to expose the brain, it is usually removed attached to the calvarium. The leptomeninges are present, but because they are so transparent, in this photograph they are barely visible on the surface of the caudal half of the brain between gyri. B, Spinal cord, horse. The dura mater is the thick opaque layer dissected from and lying to the right of the spinal cord. The leptomeninges (pia-arachnoid layer) are present (but not readily visible in this photograph) on the exposed surface of the spinal cord. Arrows indicate spinal nerve roots. (Courtesy Dr. J.F. Zachary, College of Veterinary Medicine, University of Illinois.)Stay updated, free articles. Join our Telegram channel
Full access? Get Clinical Tree
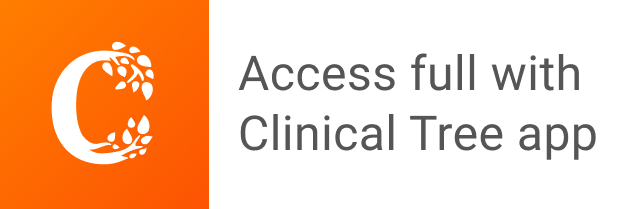