4 Pierre-Louis Toutain Pharmacokinetics (PK) studies the fate of drugs in the animal whereas pharmacodynamics (PD) studies the action of drugs from its interaction with receptors, to the effect on animal populations. Pharmacokinetic/pharmacodynamic (PK/PD) integration consists of describing and explaining the time course of the drug effect (PD) via the time course of its concentration in the plasma (PK). For a systemic drug, a tenet in pharmacology is that the plasma concentration controls the concentration at the site of action (the so-called biophase where receptors, pathogens, parasites, etc. are located) and that a proportional relationship exists between the plasma and biophase concentrations at equilibrium. This is why plasma concentration, which is easy to measure, can be used as an explicative variable of the in vivo drug effect when integrating PK and PD data in a PK/PD modeling approach (Figure 4.1). Plasma concentration, unlike the biophase concentration, is also easy to control with an appropriate dosage regimen and one of the main goals of PK/PD analysis is to precisely determine the dosage regimen (dose, dosage interval). The ultimate goal in controlling the plasma drug concentration (also termed drug exposure or internal dose) is to achieve some expected endpoint in terms of drug efficacy and safety. Figure 4.1 Dose–effect relationship versus PK/PD modeling. Both approaches aim at documenting the same relationship between dose and drug response. A dose–effect relationship is a black-box approach in which the dose is the explicative variable of drug response. In a PK/PD approach, the black box is opened, thereby enabling the two primary processes that separate dose from response to be recognized. In the first step (PK), the dose is transformed into a plasma concentration profile. In the second step (PD), the plasma concentration profile becomes the variable, which explains the drug response. The difficulty with the PK/PD approach is that the development of effect and plasma concentrations over time is usually not in phase. This means that a hysteresis loop is observed when the response is plotted against plasma concentrations and data modeling is required to estimate the PD parameters (Emax, ED50, and slope). The first part of this chapter addresses the question of drug pharmacodynamics with special emphasis on the relationship between drug concentration and intensity of action at the receptor level. The second part of this chapter is devoted to the in vivo situation and to the whole drug response. The classical dose-titration methods for dose determination and their limits are described with special emphasis on the alternative PK/PD approaches. The use of PK/PD modeling in studies of veterinary drugs has been reviewed (Riviere and Toutain, 2011; Toutain, 2002; Toutain and Lees, 2004) for antibiotics (Lees et al., 2006; McKellar et al., 2004; Toutain et al., 2002), angiotensin converting enzyme (ACE) inhibitors (Toutain and Lefebvre, 2004) and nonsteroidal antiinflammatory drugs (NSAIDs) (Lees et al., 2004a; Lees et al., 2004b). A glossary of pharmacodynamic terms and symbols is given in (Lees et al., 2004a; Neubig et al., 2003). Most drugs act via an interaction with certain proteins either of the host or of the pathogen (Figure 4.2). Detailed discussions of drug mechanism of action are discussed in the specific chapters of this text. Exceptions are drugs in which the activity is based on physical properties, such as osmotic diuretics (e.g., mannitol) and antacids. Protamine that can be injected as an antidote of heparin acts as a physical antagonist by binding to it. Tiludronic acid is a biphosphonate used to prevent or to treat a variety of bone conditions. It binds to hydroxyapatite crystals and inhibits hydroxyapatite breakdown, suppressing bone resorption (Drake et al., 2008). General anesthetics were previously thought to produce their effect by simply dissolving in the lipid bilayer of the nerve membrane. It is currently acknowledged that all anesthetics act by either enhancing inhibitory signals or by blocking excitatory signals (for a review see Garcia et al., 2010). For intravenous anesthetics such as propofol and inhaled anesthetic as isoflurane, the target has been identified as the GABAA receptor (GABAR), the most abundant fast inhibitory neurotransmitter receptor in the central nervous system. Ketamine and nitrous oxide inhibit ionotropic glutamate receptors, with the strongest effects being seen on the NMDA receptor subtype. Figure 4.2 Mechanisms of drug action. For most drugs, the drug action is mediated by some biological interaction with a macromolecule of the cell, often a protein. Different proteins are involved as drug targets and the term receptor is only used when the interaction triggers a cascade of events for signal transmission. Four types of protein are targeted by drugs: enzymes, carriers, ion channels, and receptors (Figure 4.2). The term receptor should be reserved for regulatory proteins that play a role in intercellular communication. Thus, enzymes, ion channels, and carriers are not usually classified as receptors. Enzymes such as cyclooxygenases are the target site for NSAIDs and their inhibition leads to the suppression of proinflammatory prostaglandins. Acetylcholine esterase, an enzyme that metabolizes acetylcholine at the receptor site, is a target site for cholinesterase inhibitors (neostigmine, physostigmine, etc.). Cholinesterase inhibitors act indirectly by preventing the enzyme from hydrolyzing acetylcholine. Other examples of enzymes serving as drug targets are dihydrofolate reductase for trimethoprim (an antibacterial) and angiotensin converting enzyme (ACE) for ACE inhibitors such as benazepril and enalapril. Antibiotics may act by inhibiting enzymes involved in cell wall biosynthesis, nucleic acid metabolism and repair, or protein synthesis, which is why antibiotics are generally more efficacious on multiplying bacteria. Carriers (also termed membrane transport proteins) are target sites for many drugs. The Na+/K+/2Cl− symport in the nephron is the site of action of furosemide and other loop diuretics such as torasemide. Furosemide acts at the luminal surface of the thick ascending limb of the loop of Henle to prevent sodium chloride reabsorption. For all diuretics other than spironolactone, the biophase is urine, not plasma, and they need to gain access to the lumen of the nephron to develop their diuretic action. This explains why in renal failure, the dose often needs to be substantially increased, not decreased as for other drugs. ATP-powered ion pumps such as the sodium pump (Na+/K+ ATPase) are the target sites for cardioactive digitalis and the Na+/H+ pump in the gastric parietal cell is the target site for proton pump inhibitors such as omeprazole. Some drugs, such as local anesthetics, produce their effects in the nervous system and in the heart (Grace and Camm, 2000; Sills, 2011) by directly interacting with ion channels. They inhibit voltage-gated Na+ channels in sensory neurons by binding to specific sites within the Na+ channel and produce a direct effect by incapacitating the protein molecule. Their affinity varies with the gating state of the channel, with a high affinity when the channels are opened and inactivated during action potentials at high frequency, as occurs during pain or a cardiac arrhythmia. Most antiepileptic drugs act on voltage–gated Na+ channels and voltage-dependent gated calcium channels are the main target of most calcium-channel blockers, such as the antiarrhythmic drugs verapamil and amlodipine. This mechanism of drug action on ion channels should not be confused with that of ligand-gated ion channels, which function as ionotropic receptors (see Section Macromolecular Nature of Drug Receptors). Other nonreceptor/nonprotein targets as sites of action are nucleic acids for drugs such as actinomycin D, an antineoplastic antibiotic. DNA is also the target for a number of antibiotics (quinolones) as well as mutagenic and carcinogenic agents. A receptor is a molecule or a polymeric structure on the surface of or inside a cell that specifically recognizes and binds an endogenous compound. Binding sites are three-dimensional structures, forming pockets or grooves on the surface of protein that allow specific interactions with compounds known as ligands, which are molecules of complementary shape to the protein binding site (lock and key analogy). Receptors possess an effector system (also termed signal-transduction pathways). In this they differ from acceptors which are molecules without signal-transduction pathways (e.g., serum albumin), characterized by a binding process that is not followed by a physiological response. Endogenous neurotransmitters, such as hormones, act as molecular messengers and are endogenous ligands. Drugs may be viewed as exogenous ligands. After attachment to a receptor site, a drug may produce a cascade of biochemical events that result in drug action. A drug is said to be an agonist when it produces a measurable physiological or pharmacological response characteristic of the receptor (contraction, relaxation, secretion, enzyme activation, etc.). If a drug binds to the same site as the endogenous ligand, the drug is said to be a primary agonist, in comparison to an allosteric agonist which binds to a different region of the receptor. Most anesthetic drugs allosterically modulate GABAR and disrupt corresponding physiological circuits. A full agonist produces a maximal effect under a given set of conditions whereas a partial agonist produces only a submaximal effect regardless of the amount of drug applied. For opioid receptors, morphine and fentanyl are full agonists, able to initiate strong analgesia, while buprenorphine is a partial agonist (Lees et al., 2004b). Even if buprenorphine is unable to achieve the same level of analgesia provided by a maximally effective dose of full agonists, it may be preferred for postsurgical analgesia because its effect is of long duration and adverse effects are minimal. In contrast to an agonist, some drugs may be unable to trigger any action on their own, after attachment to a receptor site, but are able to block the action of other agonists. These “silent drugs” are termed antagonists; most drugs used in therapeutics are receptor antagonists and prevent the action of natural agonists (neurotransmitters, hormones, etc.). Some drugs may be both agonist and antagonist, for example butorphanol, a central-acting opioid analgesic, is mainly an antagonist at the mu receptor but is an agonist at the kappa receptor. Two forms of antagonism can be distinguished: competitive and noncompetitive antagonism. In competitive antagonism, antagonists act on the same receptor as the agonist; this is said to be reversible when it can be surmounted by increasing the concentration of the agonist. Examples of therapeutic agents acting by competitive antagonism are atropine (an antimuscarinic agent) and propranolol (a beta blocker). In irreversible (competitive) antagonism, a net displacement of the antagonist from its binding site cannot be achieved by increasing the agonist concentration and, operationally, it resembles noncompetitive antagonism. This occurs when the antagonist is bound covalently and irreversibly to its receptor binding site. Although there are few drugs of this type, irreversible antagonists are used as experimental probes for investigating receptor function. Noncompetitive antagonism refers to the situation where a drug blocks the cascade of events, normally leading to an agonist response, at some downstream point. This occurs with Ca2+ channel blockers, such as nifedipine, which prevent the influx of calcium ions through the cell membrane and nonspecifically block any agonist action requiring calcium mobilization, as in smooth muscle contraction. The concept of physiological antagonism refers to the interaction of two drugs whose opposing actions on a physiological system tend to cancel each other out. For example, histamine acts on receptors of the parietal cells of the gastric mucosa to stimulate acid secretion, while omeprazole blocks this effect by inhibiting the proton pump. Antagonists were viewed solely as “silent ligands” until the discovery of the so-called inverse agonists. An inverse agonist is a drug that acts at the same receptor as that of an agonist, yet produces an opposite effect (see Section Drug Receptor Theories: from the Occupancy Theories to the Two-State Model for the mechanism of inverse agonist action). Figure 4.3 summarizes the spectrum of activities that ligands can display. Figure 4.3 Schematic representation of the spectrum of drug effect from full agonist (maximum positive effect) to full inverse agonist (maximum negative effect). Such a spectrum is only seen if a constitutive receptor activity exists which can be inhibited by an inverse agonist (see Figure 4.9 for explanation). According to the “receptor theory”, drugs may be agonists or antagonists. A full agonist produces full receptor activation leading to a maximal response; a partial agonist produces a submaximal response and possible blockade of a full agonist activation. An antagonist does not produce any physiological response but is able to block the response of an endogenous ligand or exogenous agonist. In the framework of the two-state model, any drug is viewed as an agonist, and an antagonist is a neutral agonist producing no response. According to this model “efficacy” is genuinely explained by the relative affinity of the drug for one of the states of the receptor (activated or resting state) and inverse agonists are drugs showing selectivity for the resting state of the receptor. An inverse agonist acts as an antagonist but has the supplementary property over a classical antagonist of reducing receptor-mediated constitutive activity. The concentration–effect relationship is determined by two features of the drug–receptor interaction, namely drug affinity and drug efficacy. The affinity of a drug is its ability to bind to a receptor. Affinity is determined by the chemical structure of the drug and minimal modification of the drug structure may result in a major change in affinity. This is exploited to discover new drugs. Affinity determines the concentration of drug required to form a significant number of drug–receptor complexes that in turn are responsible for drug action. The numerical representation of affinity for both an agonist and an antagonist is the constant of affinity, denoted by Ka (dimension M−1, i.e., liter per mole). A Ka of 107M−1 means that one mole of the ligand must be diluted in 107 liters of solvent to obtain a concentration of the free ligand able to saturate half the maximal binding capacity of the system. The reciprocal of Ka is the equilibrium dissociation constant of the ligand–receptor complex, denoted by Kd (dimension M i.e., mole per liter). A Kd of 10−7M means that a free ligand concentration of 10−7 mole per liter is required to saturate half the maximal binding capacity of the system. The lower the Kd value of a drug, the higher the affinity for its receptor. Radioactive receptor ligands (radioligands) or fluorescent probes are used to accurately determine receptor affinity. The relationship between bound and free ligand may be described by a hyperbolic equation (Figure 4.4) corresponding to the Michaelis Menten equation: Where Bmax (a parameter) represents the maximal binding capacity (the total number of receptors), Free is the molar concentration of the free ligand, Bound is the bound ligand concentration and Kd (a parameter) is the equilibrium constant of dissociation. Figure 4.4 Relationship between free drug concentration (Free) (independent variable), that is the driving concentration, and receptor-bound drug (dependent variable). Affinity of a drug for its receptor is expressed by Kd (a low Kd means a high affinity). Bmax indicates the maximal binding capacity of the receptor. From this equation, the receptor occupancy (i.e., the fraction of receptor occupied) can be described by: This is known as the Hill–Langmuir equation. The generation of a response for the drug–receptor complex is governed by a property named efficacy. Efficacy is the drug’s ability, once bound, to initiate changes that lead to the production of responses. It is a property of the ligand/receptor pair. This term is used to characterize the level of maximal response (Emax) induced by an agonist. In contrast, a pure antagonist has no intrinsic efficacy because it does not initiate a change in cell functions. This concept of efficacy is not to be confused with the drug’s clinical efficacy whereby an antagonist may be fully efficacious. This is because blocking the binding of an endogenous agonist to the receptor by an antagonist may be clinically useful. Potency corresponds to the concentration of drug required to achieve a given effect. It is expressed by the EC50 (or the IC50 if the effect is an inhibition), that is the concentration of an agonist which produces 50% of the maximum possible response for that agonist (Figure 4.5). Potencies of drugs vary inversely with the numerical value of their EC50 and the most potent drug is the one with the lowest EC50. Figure 4.5 The dose–response relationship and definition of the three main pharmacodynamic parameters. The concept of drug efficacy and potency is also used in a clinical context. Drug efficacy is the property of most interest to clinicians who are looking for the most efficacious drug. For example, it was shown using an inflammatory model in horses that flunixin was more efficacious than phenylbutazone in induced lameness (Toutain et al., 1994). For some drugs such as loop diuretics, there is actually a maximum possible effect that can be obtained regardless of how large a dose is administered and this is termed the high ceiling effect. When different drugs within a series are compared, the most potent drug is not necessarily the most clinically efficacious (Figure 4.6). For example, butorphanol is more potent but less efficacious than morphine for analgesia. Another example is glucocorticoids. All glucocorticoids are able to totally suppress the endogenous cortisol secretion (adrenal suppressive effect), as well as to ensure a full eosinopenia, thymus involution and in regard to these suppressive endpoints, they all have the same pharmacological efficacy. However, their potencies are very different, with dexamethasone, betamethasone, and triamcinolone acetonide being 20 to 30-fold more potent than cortisol, whereas prednisolone and methylprednisolone are only four to fivefold more potent than cortisol. A low potency is only a disadvantage when the effective dose is too large to be convenient; for example for a spot-on, eye drop or intraarticular administration, the volume to be administered must be small and only relatively potent drugs can be administered in this way. Figure 4.6 Drug potency versus drug efficacy. These two terms are often confused. Potency is expressed in terms of concentration (X axis) and EC50 is the parameter measuring drug potency. Efficacy (Y axis) expresses the level of response with a maximal possible effect (Emax). Efficacy is the parameter of interest for a clinician but potency may be a limiting factor if the drug has to be administered in a small volume. Here drug A has a higher potency than drug B but has a lower efficacy. The drug receptor interaction is responsible for the specificity and selectivity of drug action. When the drug acts only on a single target (enzyme, receptor, etc.), it is said to be specific. Specificity is linked to the nature of the drug–receptor interaction and more precisely to the macromolecular structure of the receptors (or enzymes). As receptors are generally proteins, the diversity in three-dimensional shape required for ligand specificity is provided by the polypeptide structure. The recognition of specific ligands by receptors is based on the complementarity of the three-dimensional structure of the ligand and a binding pocket on the macromolecular target. The shapes and actions of receptors are currently being investigated by X-ray crystallography and computer modeling. Specificity is rare. Most drugs can display activity towards a variety of receptors and are more often selective than specific. For example histamine antagonists produce several effects, such as sedation and prevention of vomiting, which do not depend on histamine antagonism. Selectivity is related to the concentration range. The drug may be specific at a low concentration if it activates only one type of target, whereas several targets may be involved simultaneously (activated, inhibited, etc.) if the drug concentration is increased. This is the case with NSAIDs and inhibition of the different subtypes of cyclooxygenases (COX-1 vs. COX-2 isoenzymes) (Figure 4.7). Cortisol possesses both glucocorticoid and mineralocorticoid properties and cortisol at pharmacological dose causes unwanted side effects, such as fluid–electrolyte imbalance, which is the reason why it cannot be used as an antiinflammatory drug. This is due to the close structural relationship of the nuclear glucoreceptor (GR) and the mineralocorticoid receptor (MR). The in vivo natural ligand of the MR is aldosterone. A biological paradox is that the affinity for the MR is similar for aldosterone and cortisol, while cortisol concentration in epithelial tissues is much higher (100–1000 fold) than aldosterone concentration, raising the issue of a possible unwanted binding of cortisol to the MR. Actually, the in vivo selectivity of aldosterone for the MR exists despite its much lower concentration and is of enzymatic origin; it is determined by the intracellular metabolism of cortisol into cortisone, an inactive metabolite with a low affinity for the MR. This inactivation of cortisol is carried out by the unidirectional 11β-hydroxysteroid dehydrogenase (11βHSD) of type 2, an enzyme expressed in the epithelial aldosterone target cell. 11βHSD can be inhibited by various substances, such as furosemide and licorice. For synthetic glucocorticoids, it was desirable to separate the gluco- from the mineralocorticoid effect and selectivity for the GR (associated to the antiinflammatory effect) was obtained by structural modification of the cortisol; first an increase of the selectivity was obtained by the introduction of Δ1-dehydro configuration (as for prednisolone) and then by adding a hydrophobic residue in position 16 as a 16-α-methyl group for dexamethasone or a 16-β-methyl group for betamethasone and beclomethasone(Farman and Bocchi, 2000). Figure 4.7 Drug selectivity. For a non steroidal antiinflammatory drug (NSAID) such as nimesulide, the antiinflammatory properties are linked to the inhibition of cyclooxygenase (COX). There are two isoforms of COX, namely COX-1 and COX-2. COX-2 is an inducible enzyme formed at the site of inflammation and producing proinflammatory prostaglandins such as PGE2, whereas COX-1 is a constitutive enzyme that performs a range of housekeeping functions. The selective inhibition of COX-2 is considered the primary action to be achieved for a NSAID and the nonselective inhibition of COX-1 is considered detrimental, especially for the digestive tract (ulceration). This COX-2/COX-1 selectivity can be explored ex vivo using a whole blood assay (Toutain et al., 2001b). When this assay was conducted using canine blood, the production of TxB2 by platelets was used to measure COX-1 inhibition and the production of PGE2 from LPS-stimulated leukocytes was used to measure COX-2 inhibition. The IC50 values were 1.6±0.4 μM for COX-2 and 20.3±2.8 μM for COX-1, indicating that nimesulide is about 12 times more potent for COX-2 than for COX-1 isoenzymes. Nevertheless, nimesulide cannot be considered as a selective COX-2 inhibitor but only as a preferential COX-2 inhibitor. COX-2 would need to be almost totally inhibited to obtain a full antiinflammatory effect and this is impossible to achieve without any COX-1 inhibition. Source: Toutain 2001b. Reproduced with permission of John Wiley & Sons, Inc. Lack of selectivity in vivo may lead to an overall complex and unpredictable pharmacological response and even undesirable side effects. This is the case, for example, for drugs administered as racemic mixtures of stereoisomers. Stereoisomers can exhibit not only very different potency and efficacy for a given effect, but also very different dispositions with possible interconversion between stereoisomer, rendering it difficult to predict the net effect of the drug and its time development. For antibiotics, antiparasitics, and similar drugs, the concept of selectivity is twofold: selectivity regarding the host versus selectivity regarding the target. Pathogen selectivity is generally expressed in terms of the spectrum of activity using an classification tool such as the staining properties (Gram positive vs. Gram negative) or a taxonomical classification (family, species, strains, etc.). Selectivity regarding the host is also an important characteristic to minimize the toxicity of antibiotics and insecticides. For example, penicillin inhibits key enzymes (transpeptidases, carboxypeptidases) involved in the peptidoglycan biosynthesis required for synthesis of the bacterial wall. These enzymes do not exist in mammals, ensuring an excellent selectivity and safety of this class of antibiotics in mammals. Assessing the therapeutic usefulness of a new drug requires the determination of its full spectrum of biological activities towards a variety of relevant targets. The next task is then to explore this in vivo, and select an appropriate dosage regimen that maintains the plasma drug concentration within the range in which only the desired response is expressed (i.e., within the so-called therapeutic window). Several chemical intermolecular forces such as ionic bonds, hydrogen bonds, and Van der Waals forces may be involved in reversible binding of the drug to the receptor. In contrast, drug–receptor interactions involving covalent bond formation (very tight) are generally irreversible. Covalent binding to receptors is relatively rare. However, covalent binding is more frequent for drugs acting on enzymes. COX-1 inhibitors are generally reversible, although aspirin acts as a noncompetitive inhibitor of platelet COX-1 through a covalent binding mechanism. This is achieved by irreversible acetylation and explains the duration of the action of aspirin on blood coagulation. As platelets have no nucleus, the effect of aspirin is reversed by the production of new platelets. Omeprazole (a proton pump inhibitor) is another example of irreversible binding to an enzyme (H+,K+-ATPase). Drugs that bind covalently to DNA (alkylating agents) are extensively used as anticancer drugs. Since many drugs contain acid or amine functional groups that are ionized at physiological pH, ionic bonds are formed by the attraction of opposite charges in the receptor site. Ionic bonds are the strongest noncovalent bonds. The attraction of opposite charges is brought about by polar–polar interactions as in hydrogen bonding. Although this electrostatic interaction is weaker than the ionic bond, an important feature of hydrogen bonding is the structural constraint. Thus the formation of hydrogen bonds between a drug and its receptor provides some information about the three-dimensional structure of the resulting complex. The same forces are responsible for the shape of the protein and for its binding properties, so shape influences binding and, in turn, binding can influence protein shape. The ability of protein to change shape is called allostery. Many receptors are transmembrane proteins embedded in the lipid bilayer of cell membranes. They have two functions: ligand binding and message transduction. Transmembrane receptors include metabotropic and ionotropic receptors (Figure 4.8). The four superfamilies of receptor proteins include: (i) ligand-gated ion channels, which are membrane-bound receptors, directly linked to an ion channel; (ii) G-protein coupled receptors, which are membrane-bound receptors coupled to G-proteins; (iii)tyrosine kinase-linked receptors, which are membrane bound receptors containing an intrinsic enzymatic function (tyrosine kinase activity) in their intracellular domain; and (iv) transcription factor receptors that are intracellular receptors regulating gene transcription. Figure 4.8 Types of receptors and signaling mechanisms. The activation of metabotropic receptors (tyrosine kinase receptors and G-protein-coupled receptors) leads to some changes in metabolic processes within the cell, whereas ionotropic receptors directly open or close an ion channel. When an ionotropic receptor is activated, it opens a channel that immediately allows ions such as Na+, K+, or Cl− to flow. In contrast, when a metabotropic receptor is activated, a series of intracellular events is first triggered that may also subsequently result in ion channel opening. G-protein-coupled receptors (GPCRs), also known as seven transmembrane receptors (7TM receptors), transduce an extracellular signal (ligand binding) into an intracellular signal (G-protein activation). The signal is transferred via conformational alterations to a member of the family of G-proteins. G-proteins may act directly on an ionic channel or activate an enzymatic system (adenylyl cyclase, guadenylyl cyclase, phospholipase C, etc.) to release a range of second messengers (cAMP, cGMP, IP3, etc.), which ultimately permits certain ions to enter or leave the cell. Muscarinic acetylcholine receptor, adrenoceptors (α1, α2, β), dopamine, histamine, opioids, ACTH, and other receptors are examples of 7TM receptors. The so-called receptor tyrosine kinase (RTK) family is also a class of metabotropic cell surface receptors, which exert their regulatory effect by phosphorylating different effector proteins. An RTK consists of an extracellular binding site and an intracellular portion with enzymatic activity (tyrosine kinase, serine kinase) and usually spans the cell membrane once only. Tyrosine kinase enzymes can transfer a phosphate group from ATP to a tyrosine residue in an intracellular protein to increase its phosphorylation. Protein phosphorylation is one of the underlying mechanisms regulating protein function. It can alter the biological properties or the interaction of proteins with other proteins or peptides. This family of receptors includes insulin, IGF-1, cytokines, and epidermal growth factor. The hormones and growth factors that act on this class of receptors are generally growth promoting and function to stimulate cell division. Ionotropic receptors activate transmembrane ion channels. They contain a central pore, which functions as a ligand-gated ion channel. Nicotinic cholinergic receptor, GABAA receptor, and glutamate, aspartate, and glycine receptors are ligand-gated ion channels. The most important veterinary antiparasitic drugs (ivermectins, fipronil) act on ligand-gated anion channels and imidacloprid acts on a ligand-gated cation channel (Raymond and Sattelle, 2002). For ivermectin, glutamate-gated chloride channels have been localized to the pharynx of Caenorhabditis elegans and it was suggested that ivermectin could inhibit the pharyngeal pumping in Haemonchus contortus that is required for its feeding (Forrester et al., 2004). Transcription factor receptors such as those for steroid hormones, thyroid hormone, vitamin D, or retinoids receptors are intracellular proteins serving as transcription factors. After binding the ligand, for example a steroid hormone, the activated receptors translocate to the nucleus and bind to a DNA sequence called the response element and initiate the transcription of specific gene(s). Endogenous neurotransmitters (acetylcholine, norepinephrine, etc.) often bind to more than one type of receptor. This allows the same signaling molecule to produce a variety of effects in different tissues. For example acetylcholine acts via both G-protein receptors (e.g., M2 muscarinic receptors in the heart) and ligand-gated channels (nicotinic muscle receptors). Historically, the classification of receptors has been based on their effect and relative potency towards selective agonists and antagonists. Now the association of molecular biology and cloning techniques has led to the discovery of novel receptor subtypes and their expression as recombinant proteins has facilitated the discovery of more selective drugs. The International Union of Basic and Clinical Pharmacology (IUPHAR) has a committee on receptor nomenclature and drug classification and regularly publishes updated reviews and a compendium in its Journal (Pharmacological Reviews; see www.iuphar.org) (Neubig et al., 2003). Drug receptor theories consist of a collection of evolving models that correspond to the historical progression of knowledge and permit qualitative and quantitative description of the relationship between drug concentrations and their effect. Before the advent of molecular biology, the various effects of agonists and antagonists were described by operational mathematical modeling, that is with no or minimal mechanistic considerations (a black-box approach). Effects were measured on isolated tissue in an organ bath chamber and the collected data were modeled using relatively simple equations derived from the law of mass action, as in Equation 4.1. Occupancy Theory was the first model and was proposed by Clark in 1923 (Rang, 2006). The receptor–ligand interaction was described as a bimolecular interaction and the receptor–ligand complex was considered responsible for the generation of an effect. This model assumes that the drug response is a linear function of drug occupancy at the receptor level. Consequently, the drug has to occupy all receptors to achieve a maximal effect (Emax), and the response is terminated when the drug dissociates from the receptor. Thus, in the equation for receptor binding (see Equation 4.1) the terms B and Bmax can be replaced with E (effect) and Emax, leading to:
Mechanism of Drug Action and Pharmacokinetics/Pharmacodynamics Integration in Dosage Regimen Optimization for Veterinary Medicine
Introduction
Types of Drug Targets
Drug Receptor and Ligand as Agonist or Antagonist
Drug Affinity, Efficacy and Potency
(A) When the drug response (arithmetic Y axis) is plotted against the tested dose (arithmetic X axis), a hyperbolic relationship is often observed with a maximal effect denoted Emax. EC50 is the concentration that produces an effect equal to half Emax.
(B) When the same data are represented using an X log scale, the relationship becomes sigmoidal with a more or less steep slope. The advantage is to compress the dose scale and to visualize the slope more easily. A sigmoidal curve allows the definition of three drug parameters, namely Emax, ED50, and the slope (ΔY/ΔX). Emax describes the drug efficacy and EC50 describes the drug potency. The slope (measured by the n of the Hill equation; see Equation 4.4 in the text) indicates the sensitivity of the dose–effect curve. The slope of the curve is involved in the drug selectivity (see Figure 4.11).
(C) This plot shows that the concentration effect curve is situated to the left of the free concentration (occupancy)-bound concentration curve with EC50 generally ≪ Kd.
Drug Specificity and Selectivity
Chemical Forces and Drug Binding
Macromolecular Nature of Drug Receptors
(A) Ion channels receptors. Ion channels inhibitors or ligand-gated receptors (ionotropic receptors) are proteins forming on aqueous pores in the plasma membrane. Ligand binds at a specific site on the receptor. This leads to conformational changes, which open the channel and allow ions to flow into the cell. The change in ion concentration within the cell triggers cellular response. These receptors react in milliseconds. This type of receptor is not to be confused with voltage-gated ion channels that are not considered to be receptors (no signal transduction).
(B) G-protein-coupled receptors. G-protein-coupled receptors (GPCR) are single polypeptide chains forming seven transmembrane domains within the membrane with a segment able to interact with G-proteins.
B1 (inactive state): G-protein (GP) is attached to the cytoplasmic side of the membrane and is inactive as long as a ligand binds to GPCR. An ion channel or enzymes are possible terminal effectors of GPCR.
B2 (active state) steps 1 to 4:
Step 1: binding of a ligand changes the shape of the GPCR that interacts with a GP.
Step 2: interaction causes activation of GP, with guanosine diphosphate (GDP) being exchanged against guanosine triphosphate (GTP).
Step 3: the activated GP (Gα-GTP) moves either towards an enzyme, or directly modulates the conductance of an ionic channel (e.g., muscarinc receptor).
Step 4: when an enzyme is activated (adenylyl cyclase, guanylyl cyclase, etc.) a second messenger (Ca2+, cAMP, cGMP, etc.) is generated and can mediate an action. Then G-protein hydrolyses GTP back to GDP.
The advantage of this signal transduction pathway is twofold: signal amplification and signal specificity.
(C) Tyrosine kinase receptors
C-1 (inactive state): tyrosine kinase receptors (TKR) are transmembrane receptors consisting of individual polypeptides each with a large extracellular binding site and an intracellular tail with an enzyme (tyrosine (Tyr) kinase, serine kinase).
C-2 (active state): when a ligand binds to both receptors, the two receptor polypeptides aggregate to form a dimmer. This activates the tyrosine kinase part of the dimmer. Each uses ATP to phosphorylate the tyrosines on the tail of the other polypeptide as well as other down-stream signaling proteins. The receptor proteins are now recognized by relay proteins inside the cell. Relay proteins bind to the phosphorylated tyrosines and may activate different transduction signals.
(D) Nuclear receptors. Intracellular receptors are located in the cytoplasm or the nucleus (NO, thyroid hormones, steroids, etc.) the signal molecule (lipophilic) must be able to cross the plasma membrane. The ligand binds to a receptor called a nuclear receptor, although some are located in the cytosol (e.g., glucocorticoid receptors) and migrate to the nucleus after binding a ligand. This activation pathway leads to a long-lasting effect, usually over several hours.
Receptor Types and Subtypes
Drug Receptor Theories: from the Occupancy Theories to the Two-State Model
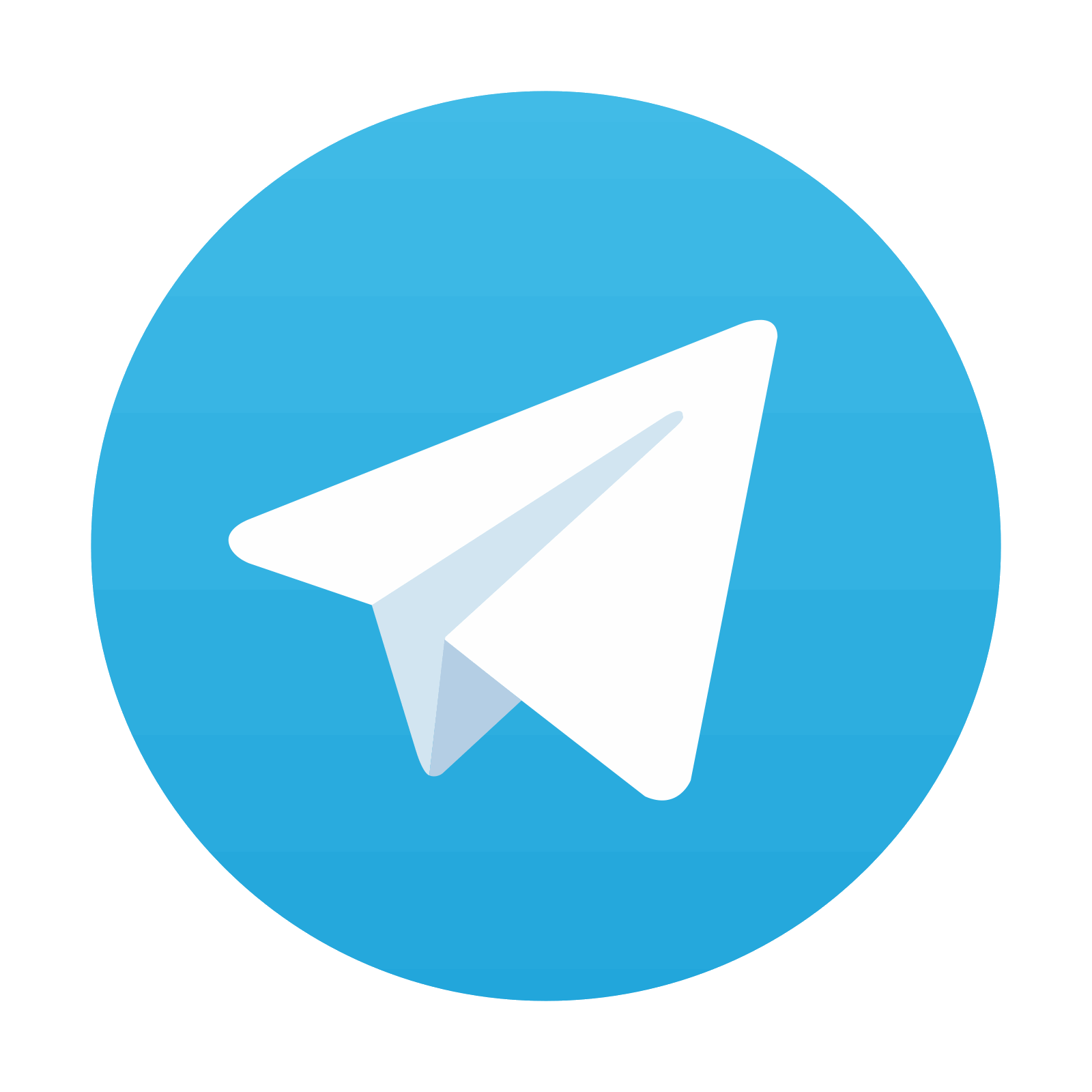
Stay updated, free articles. Join our Telegram channel
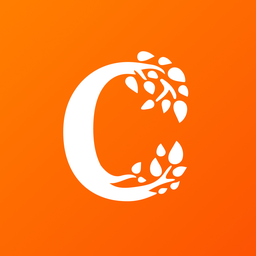
Full access? Get Clinical Tree
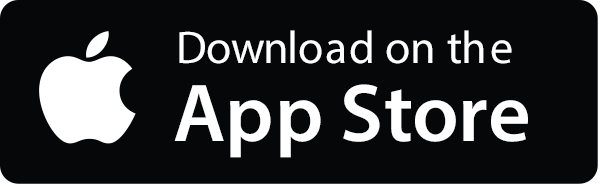
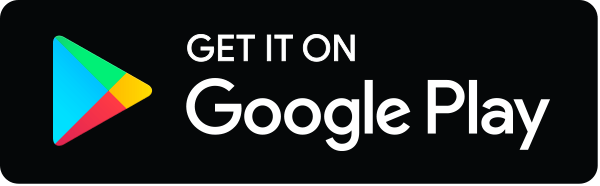