41 Carlos E. Lanusse, Fernanda A. Imperiale, and Adrian L. Lifschitz The integration of available information on the host–parasite–environment relationship with a more complete understanding of the pharmacological properties of existing antiparasitic drugs should result in more efficient parasite control in domestic animals. The time of parasite exposure to active drug concentrations determines the efficacy and/or persistence of activity for the most of the anthelmintic drugs used in veterinary medicine. The characterization of drug concentration profiles in tissues of parasite location and within target parasites provides a basis for understanding the differences in therapeutic and preventive efficacies observed for the different anthelmintic chemical families. The avermectins and milbemycins are closely related 16-membered macrocyclic lactones (MLs), produced through fermentation by soil dwelling actinomycetes (Streptomyces). It was the unique combination killing of endo- and ectoparasites that gave rise to the embracing name endectocide by which the MLs are recognized (Shoop et al., 1995a). Information on the antiparasitic properties of the different ML compounds in all animal species has been thoroughly reviewed elsewhere (Campbell, 1985; Shoop et al., 1995a; McKellar and Benchaoui, 1996). Specific literature devoted to the MLs (Vercruysse and Rew, 2002; Gonzalez-Canga, 2012), is recommended for readers interested on any further detailed issue within the topic. Enormous progress on understanding the relationship among disposition kinetics, tissue distribution, and the patterns of antiparasitic persistence for the ML molecules has been achieved, particularly in ruminant species. The purpose of this chapter is to provide an updated and integrated overview of the main basic pharmacological features of the ML compounds, which account for their outstanding long-persistent broad-spectrum antiparasitic activity. The MLs are now considered the most widely used broad-spectrum antiparasitic drugs in veterinary medicine. Chapter 43 describe MLs use as ectoparasiticides. Avermectins are produced as a mixture of different components from fermentation of Streptomyces avermitilis. These natural products are denoted as A (those containing a methoxy group at the 5-position) and B (with a hydroxy group at the 5-position). The highest anthelmintic potency is held by the B1 homologs. The avermectin family includes a series of natural and semisynthetic molecules, such as abamectin, ivermectin, doramectin, eprinomectin, and selamectin. Abamectin is the naturally occurring avermectin approved for animal use and the starting material for the production of ivermectin. In fact, ivermectin, the first marketed endectocide molecule, is a semisynthetic derivative of the avermectin family. Ivermectin is the generic name given to a mixture of two chemically modified avermectins containing at least 80% of 22-23-dihydroavermectin B1a and less than 20% of 22-23-dihydroavermectin B1b (Fisher and Mrozik, 1989). Doramectin was obtained by mutational biosynthesis in which the precursor (cyclohexanecarboxylic acid) was fed to a mutant strain of Streptomyces avermitilis (Goudie et al., 1993). Eprinomectin is a semisynthetic avermectin compound, developed for topical use in cattle (Shoop et al., 1996). Selamectin is a semisynthetic monosaccaride oxime derivative of doramectin more recently introduced into the market for parasite control in dogs and cats (Bishop et al., 2000). The main chemical differences among the avermectin-type compounds are shown in Figure 41.1. It has been shown that minor chemical changes may have a profound impact on the activity of the avermectin analogs. For instance, substitution of the C-5 hydroxy with an oxo- substituent on ivermectin or doramectin monosaccharides can drastically reduce activity against Haemonchus contortus larvae. Figure 41.1 Chemical structures of the main avermectin compounds used in veterinary medicine. The main chemical differences between abamectin (the natural fermentation product) and other avermectin-type molecules are shown. The commercially available preparations of abamectin and ivermectin contain at least 80% of the avermectin B1a (the a-component has a secondary butyl side chain at the 25-position) and no more than 20% of avermectin B1b (the b-component has an isopropyl substituent at the 25-position). Eprinomectin contains at least 90% of the B1a and no more than 10% of the B1b component. Nemadectin, moxidectin, and milbemycin oxime belong to the milbemycin family. Milbemycin oxime consists of two oxime derivatives (A4 and A3 components) of the 5-didehydromilbemycin in an 80 : 20 ratio. Moxidectin is a milbemycin compound produced by a combination of fermentation and chemical synthesis. Moxidectin is obtained by chemical modification of nemadectin, the natural compound produced when Streptomyces cyaneogriseus is grown under controlled culture conditions (Takiguchi et al., 1980). The molecular structures of the two endectocide families are superimposable (Shoop et al., 1995a); however, milbemycins do not have the C13 disaccharide substituent in the macrolide ring (Figure 41.2). Additionally, moxidectin differs from ivermectin at the 23-position, where it possess a metoxime moiety. Moxidectin also has a differential substituted olefinic side chain at the 25-position. In spite of these chemical differences, both families share some structural and physicochemical properties, and their broad-spectrum antiparasitic activity against nematodes and arthropods at extremely low dosage rates (Figure 41.2). Figure 41.2 Comparison between avermectin (ivermectin) and milbemycin (moxidectin) molecular structures. The main pharmacological features shared by compounds in both families are listed. The main chemical differences between ivermectin and moxidectin are at the 13-, 23-, and 25-positions (see text for further explanation). Various compounds from both families are used in livestock, companion animals, wildlife species, and humans (ivermectin). Abamectin, ivermectin, doramectin, and moxidectin, currently marketed as injectable, pour-on (cattle), and oral (sheep, goats) formulations, are the most commonly used MLs worldwide to control endo- and ectoparasites in livestock. Exceptional potency, high lipophilicity, and the prolonged persistence of their potent broad-spectrum activity are distinctive features among antiparasitic drugs. The broad-spectrum ecto–endoparasitic activity of the ML from both families includes selective toxic effects on insects, acarines, and nematodes. However, they do not possess efficacy against cestode and trematode parasites. The MLs induce reduction in motor activity and paralysis in both arthropods and nematodes. The paralytic effects are mediated through GABA and/or glutamate-gated chloride channels (GluCl), collectively known as ligand-gated chloride channels. MLs may act on both receptors and a putative GABA-receptor subunit has been localized along the ventral nerve cord and several neurons in the head of H. contortus (Blackhall et al., 2003). However, considerably higher ML concentrations are required for the GABA-mediated effects than for those on the GluCl channels. In fact, a high affinity binding to GluCl channels, producing a slow and irreversible increase in chloride membrane conductance (Forrester et al., 2003), hyperpolarization, and flaccid paralysis of the invertebrate somatic muscles is now proposed as a main mode of action (Martin et al., 2002; Geary, 2005). The ML endectocides cause paralysis and death of both arthropod and nematode parasites due to their paralytic effects on the pharyngeal pump (Geary et al., 1999), which affects nutrients ingestion, and on the parasite somatic musculature limiting its ability to remain at the site of predilection in the host. In addition, inhibitory effects of the MLs on the female reproductive system may explain the observed reductions in parasite egg production (Fellowes et al., 2000). In H. contortus, the pharynx appears to be the most sensitive of the affected muscles (Sheriff et al., 2005). A schematic representation of the proposed main mode of action for the ML endectocides is shown in Figure 41.3. Figure 41.3 Schematic representation of the proposed mode of action for the macrocyclic lactone endectocides. The GluCl channels are members of the ligand-gated ion channel superfamily uniquely found in invertebrates. Some channel subunits have now been cloned from different nematode parasites, including the free-living nematode Caenorhabditis elegans, H. contortus, and Cooperia spp. (Forrester et al., 2003; Njue and Prichard, 2004), which has facilitated enormous progress in the understanding of the mode of action and mechanisms of resistance of the MLs. A good correlation between the presence of GluCl on neurons and muscle cells and the electrophysiological effects induced by MLs has been observed (Martin et al., 2002). Although several GluCl channel subunits have been identified, the sensitivity to these drugs seems to be associated with the alfa-subunit of the receptor (Arena et al., 1992; Cully et al., 1994; Njue and Prichard, 2004), which is encoded by different genes indicating that nematode resistance to these compounds may be polygenic. The different ML compounds exhibit a similar, but not identical, spectrum of activity against arthropod and nematode parasites and also show differences on the persistence of their antiparasitic activity. Even though compounds in both the avermectin and milbemycin families appear to have the same mode of action, there is now evidence of some subtle pharmacodynamic differences between families, which seems to account for a higher pharmacological potency of moxidectin compared to ivermectin, particularly evident against resistant nematode parasites. These pharmacodynamic differences have been observed at the GluCl channel where moxidectin is 2.5-fold more potent than ivermectin in terms of the required EC50 to activate this receptor expressed in Xenopus oocytes (reviewed by Prichard et al., 2003). More recently, Hibbs and Gouaux (2011) proposed a model for the ivermectin binding interactions with amino acids at the GluCl channel of C. elegans. Considering the structural differences between moxidectin and ivermectin, it is likely that interaction of moxidectin with the target parasite receptor may differ from that of the avermectins (Prichard et al., 2012). In contrast to mammals, nematodes have multiple genes coding for transporter proteins, which may be essential to protect them from a broad spectrum of toxins (Prichard and Roulet, 2007). The ATP-binding cassette (ABC) transporters are a family that includes different proteins such as P-glycoprotein (P-GP), multidrug resistance proteins (MRPs), and breast cancer resistant protein (BCRP). P-GP acts as an efflux pump for ML compounds, lowering the local drug concentration achieved at the GluCl receptor site of action. Whereas 14 P-GP encoding genes have been identified in the genome of C. elegans, sequences of nine P-GPs have been described in H. contortus (Williamson and Wolstenholme, 2012). Different P-GPs have been described in other nematodes, such as O. volvulus and cyathostomins. As it was demonstrated with mammalian transporters (see Chapter 50 for further discussion), it seems that they play a similar role in modulating MLs uptake and excretion in nematodes, which may modulate the MLs concentrations reaching the target site. Many studies have shown the role of transporter proteins as a potential mechanism of drug resistance in different helminth species, as it was reviewed in detail by Lespine et al. (2011). In vitro and ex vivo studies have provided clear evidence that moxidectin has a lower affinity for P-GP compared to avermectin-type compounds (Lespine et al., 2007; Ballent et al., 2014). This enhanced ivermectin efflux may account for lower active concentrations achieved at the receptor site in comparison to moxidectin. Data demonstrate minor pharmacodynamic differences between ivermectin and moxidectin, which could explain differences in spectrum and potency, and the advantageous pattern of moxidectin efficacy against ivermectin-resistant nematodes of sheep, goats, and cattle. The clinical efficacy of the MLs is closely related to their pharmacokinetic behavior, that is the time of parasite exposure to active drug concentrations, which is required to obtain optimal and persistent antiparasitic activity. Their antiparasitic spectrum and efficacy pattern are similar; however, each compound has its own dosage limiting species. Differences in physicochemical properties may account for differences in formulation flexibility, kinetic behavior, and in the potency/persistence of their endectocide activity. Thus, even slight modifications to the disposition kinetics or to the pattern of plasma/tissue exchange may notably affect the persistence of their antiparasitic effect. An enormous effort has been devoted to characterization of the kinetics of the different MLs in different species. The work on the comparative plasma (Lanusse et al., 1997; Toutain et al., 1997) and target tissues (Lifschitz et al., 1999a, 2000; Sallovitz et al., 2003) disposition kinetics of ivermectin, doramectin, and moxidectin in cattle, the disposition of doramectin (Hennessy et al., 2000; Carceles et al., 2001) and moxidectin (Alvinerie et al., 1998a) in sheep and goats, including evidences on their metabolic stability in the digestive tract (Lifschitz et al., 2005) and involvement of lymphatic transport on intestinal absorption (Lespine et al., 2006a), have contributed greatly to the understanding of the overall ML pharmacokinetic properties. Additionally, the characterization of the plasma/milk profiles of eprinomectin in cattle and goats (Shoop et al., 1996; Alvinerie et al., 1999a), the studies on the comparative milk excretion patterns of different ML in dairy sheep, and their impact on drug residue profiles in milk-derived products (Imperiale et al., 2004a), have further contributed to the knowledge on ML kinetic behavior. The main pharmacokinetic features accounting for the persistent broad-spectrum antiparasitic activity of endectocides in ruminants are summarized in Figure 41.4. Figure 41.4 Summary of the main pharmacokinetic features accounting for the extended broad-spectrum antiparasitic persistence of the macrocyclic lactone compounds in ruminants. GI, gastrointestinal tract; P-GP, P-glycoprotein. Source: Adapted from Lifschitz et al., 2000. Plasma concentration profiles may help to predict the persistence of antiparasitic activity, particularly after parenteral administration. However, the measurement of drug concentration profiles at the site of parasite location and thus antiparasitic activity (the so-called biophase in the pharmacodynamic models of Chapter 4), permits a more direct interpretation and provides a basis for understanding the differences in therapeutic and preventive efficacies observed for MLs. The characterization of drug concentrations at the sites of parasite infection for moxidectin, ivermectin, and doramectin in cattle, and the characterization of the kinetic disposition of doramectin in fluid and particulate digesta throughout the gastrointestinal tract in sheep, represent a considerable contribution to the understanding of the comparative persistence of activity of these compounds in ruminants. The highly lipophilic MLs are extensively distributed from the bloodstream to different tissues. Their extensive tissue distribution agrees with the high availability of these drugs in different parasite location tissues such as the gastrointestinal mucosal tissues, lungs, and skin in cattle (Figure 41.5), where concentrations markedly greater than those observed in plasma were measured during 50–60 days posttreatment. A strong correlation between plasma and tissue concentration profiles has been observed after the subcutaneous administration of ML compounds. The high correlation coefficients obtained showed the degree of association between ML concentrations in the bloodstream and those measured in tissues of parasites location. Despite the high correlation observed between concentrations in plasma and in tissues/fluids, ivermectin, doramectin, and moxidectin concentrations were greater in all target tissues compared to plasma. Figure 41.5 Pattern of ivermectin and doramectin distribution to parasite location tissues in subcutaneously treated calves (0.2 mg/kg). Drug availability is expressed as area under the concentration vs. time curve (AUC) in ng.day/ml (plasma) and ng.day/g (target tissues). The correlations between drug concentrations measured in plasma and in a target tissue (skin) are shown in the right panel. r, correlation coefficient. Source: Data from Lifschitz et al., 2000. MLs are extremely effective against adult and larval stages of most gastrointestinal nematodes and Dictyocaulus viviparus in the lung in cattle, exerting a prolonged protective effect. The achievement of high drug availability in lung tissue may explain their excellent activity against lungworms. The pulmonary tract was the target tissue in which the highest doramectin concentrations were recovered in parenterally treated cattle (Lifschitz et al., 2000). The persistence of the broad-spectrum anthelmintic activity of the MLs against adult and immature gastrointestinal parasites is facilitated by their advantageous pattern of distribution and prolonged residence in the digestive mucosal tissue. Differences in the plasma pharmacokinetic profiles between doramectin and ivermectin have been reported in cattle. Following intravenous administration of an aqueous micelle formulation to determine the intrinsic kinetic features of the compounds, higher plasma concentrations and delayed elimination of doramectin compared to ivermectin were reported (Goudie et al., 1993). After subcutaneous administration of the commercially available formulations to cattle, higher plasma concentrations and extended plasma residence were observed for doramectin compared to ivermectin. The kinetic variables obtained for ivermectin and doramectin in tissues of parasite location are consistent with the described plasma kinetics data. Enhanced availability and prolonged time of residence in target tissues were observed for doramectin compared to ivermectin. The oil based formulation of doramectin, potentially coupled to a slowed metabolic rate based on the presence of the cyclohexyl group at C25 (Goudie et al., 1993), may contribute to the higher plasma and target tissues drug availability of doramectin compared to ivermectin. Moxidectin is the most lipophilic endectocide agent; its high lipophilicity results in a wide tissue distribution (volume of distribution = 13.6 l/kg) and long residence in plasma, which is clearly reflected in the tissue pharmacokinetic results obtained in cattle. Distribution of the drug into adipose tissue accounts for the larger distribution for this compound compared to other antiparasitic drugs. The concentration of moxidectin in fat after 28 days of treatment in cattle has been shown to be 90-fold higher than that detected in plasma (Zulalian et al., 1994). The large tissue distribution of moxidectin in cattle agrees with the high availability of moxidectin in the gastrointestinal mucosal tissues, lungs, and skin, with concentrations ranging between 1 and 2 ng/g at 28 days posttreatment, and with the extended detection of moxidectin concentrations >0.1 ng/g up to 58 days posttreatment in those tissues. The high Cmax values and total drug availability obtained in tissues where target parasites are located is in agreement with the extensive tissue distribution of moxidectin, and thus may be relevant in terms of antiparasitic activity against internal and external parasites. Long residence times for moxidectin (between 6.8 and 11.3 days) were obtained in the different sites of parasite location, which agrees with the extended residence of moxidectin reported in fat and in the bloodstream (Alvinerie and Galtier, 1997; Lanusse et al., 1997). Moxidectin depletion half-lives in target tissues ranged from 7.73 days (skin) to 11.8 days (intestinal mucosa) (Lifschitz et al., 1999a), which were significantly longer than those determined for ivermectin and doramectin in the same tissues. The deposit of moxidectin in adipose tissue may act as a drug reservoir that contributes to the long persistence of this molecule in the bloodstream and in different tissues of parasite location. The estimated plasma elimination half-lives for different ML compounds in cattle are shown in Figure 41.6. Drug elimination half-life values for the main host tissues where target endo- and ectoparasites are located, are also shown. Figure 41.6 Comparative mean elimination half-lives for ivermectin, doramectin, and moxidectin in plasma and tissues of parasite location after their subcutaneous administration to cattle (0.2 mg/kg). Source: Data from Lifschitz et al., 1999a, 2000. A considerable excretion of ivermectin, moxidectin, and doramectin occurs via mammary glands, which invalidate their use in dairy animals. However, topical formulations of moxidectin are currently approved for use in dairy cattle in some countries without a milk withdrawal time. Although licking behavior may drastically enhance milk residues of topically administered moxdectin, the residual concentrations reported did not exceed the permitted residual concentrations at any time posttreatment (Imperiale et al., 2009). Topical application is fully discussed in Chapter 43. In an effort to identify an ML molecule that could be used in dairy cattle, an avermectin-derivative, named eprinomectin, has been developed for topical administration to cattle. While the milk/plasma partitioning for eprinomectin in topically treated cattle falls between 0.1 and 0.2 (Shoop et al., 1996; Alvinerie et al., 1999a), the milk/plasma ratio for ivermectin is between 1 (goats and cattle) (Alvinerie et al., 1993; Alvinerie et al., 1996) and 2 (sheep) (Imperiale et al., 2004b). The high lipid content of the milk may explain the higher rate of ivermectin transfer into sheep’s milk. The partitioning of moxidectin into sheep’s milk is higher than that of ivermectin with milk/plasma concentration ratios between 10.0 and 18.0 (Imperiale et al., 2004a, 2004b). This is consistent with the higher lipophilicity of moxidectin compared with ivermectin. Eprinomectin, in addition to its antiparasitic potency and broad spectrum, has a substantially reduced distribution to milk, compared to other MLs, which is probably based in some minor changes introduced to its chemical structure. In fact, changes to the milk partitioning coefficients in lactating dairy animals were achieved by manipulation of the avermectin molecule. The C4′′-epi-amino analogues unsaturated at C22-23 showing the lowest distribution into milk was eprinomectin, 4′′-epi-acetylamino-4′′-deoxyavermectin B1 (see Figure 41.1), and was the best performing compound in terms of broad-spectrum antiparasitic activity. Although some metabolic products have been recovered in plasma after administration of different MLs to cattle, these molecules are minimally metabolized in sheep and cattle, with bile and feces the major routes of excretion for unchanged parent drugs. The major liver metabolite of ivermectin in cattle is a 24-hydroxy-methyl-dihydroavermectin B1a derivative (Chiu et al., 1987), identical to that produced by steer and rat microsomes in vitro (Miwa et al., 1982). Similarly, a doramectin metabolite, accounting for 5.75% of the total recovered parent drug, was detected in plasma between 8 hours and 45 days postadministration of doramectin. The metabolism of moxidectin has been shown to produce the C29-30 and the C14 monohydroxymetyl derivatives as the main metabolic products. These and other hydroxylated metabolites have been recovered from different tissues of moxidectin-treated cattle (Zulalian et al., 1994). More extensive biotransformation of moxidectin, compared to ivermectin and doramectin, would agree with the higher ratio of metabolites/parent drug recovered in plasma of moxidectin-treated animals. Interestingly, while 90% of the fat residue at 21 days posttreatment is moxidectin parent drug, ivermectin parent compound accounts only for an 18% of the total fat residue at the same time posttreatment in cattle. The avermectins are excreted in high concentration in bile and feces of treated cattle. Following an intraruminally administered dose of doramectin in sheep, 25% is secreted in bile, with 20% of biliary metabolites enterohepatically recycled (Hennessy et al., 2000). Consistently, high concentrations of unchanged moxidectin are excreted by bile and feces (Zulalian et al., 1994), with concentrations greater than 2 ng/ml (ng/g) recovered both in bile and feces up to 48 days posttreatment in cattle (Lifschitz et al., 1999a). As pointed out by Hennessy (2000), the extent to which the biliary-secreted drug and metabolites are presented to the gut lumen, reabsorbed as free compound, and subsequently participate in enterohepatic cycling is a major contributor to parasite exposure. See Chapter 2 for an introduction to this process. The MLs abamectin, ivermectin, and moxidectin (Pouliot et al., 1997; Dupuy et al., 2001), among many other substances, have been shown to be substrates of the P-GP transport protein. In the mammalian host, P-GP participates in the mechanism of active biliary and intestinal secretion of different molecules from the bloodstream to the gastrointestinal tract. The transepithelial intestinal secretion plays a major role in the elimination of ivermectin in the rat (Laffont et al., 2002). Ivermectin clearance from the small intestine accounted for 27% of the total drug clearance, while bile secretion accounted for only 5% of the total clearance in the rat. The MLs induce a paralytic effect on the pharynx and somatic musculature of nematodes. Different in vitro assays have been used to evaluate ML effects on nematodes. The migration inhibition assay (LMIA) can be used to detect effects on somatic muscle and related neuromuscular sites. On the other hand, the larval development assay (LDA) is not specific for a particular organ and may involve pharyngeal and/or somatic muscle (Demeler et al., 2013a). A different potency among MLs has been shown with the LDA. Abamectin and moxidectin were the most potent compounds against susceptible and resistant H. contortus and H. placei strains (Kotze et al., 2014). Ivermectin concentrations of 1 nM paralyze the pharynx of H. contortus (Geary et al., 1993) and inhibit larval development of trichotrongyloid nematodes (Gill and Lacey, 1998), while much higher concentrations of ivermectin are needed to obtain an inhibitory effect of larval motility (Gill et al., 1991). Considering that 1 nM of ivermectin equals 0.87 ng/g, it may be possible to establish a correlation between the concentrations used in these in vitro assays with those obtained in target tissues after in vivo treatment. Therefore, it could be assumed that the period of time during which drug concentrations are above 1 ng/g in target tissues would be indicative of the period during which a paralytic effect on nematode pharyngeal pump occurs. The ML concentrations required at the site of parasite location to inhibit parasite establishment or development have not been determined. However, it has been theoretically assumed that the period of time during which drug concentrations are above 1 ng/g (the ivermectin concentration equivalent to that required to paralyze the pharynx of H. contortus in different in vitro pharmacodynamic assays) in target tissues, would be indicative of the period during which the in vivo anthelmintic activity may persist. While ivermectin concentrations were >1 ng/g in gastrointestinal mucosal tissue and lung tissue for 18 days posttreatment, doramectin and moxidectin profiles remained above that level for 38 days postadministration (Lifschitz et al., 2000). The comparison between these time periods and those obtained in different persistence efficacy trials is interesting. The increases of fecal eggs counts after ivermectin and doramectin administration to cattle indicate that reinfection begins 2–3 weeks and 3–4 weeks after each treatment, respectively (Ranjan et al., 1997). The characterization of drug concentrations in target tissues is useful to estimate the period of time posttreatment in which the endectocide levels at the site of parasite location remain efficacious. ML compounds have a broad spectrum of activity against adult and larval forms of many gastrointestinal nematodes in cattle. However, this pattern of efficacy varies among different nematode parasites and Cooperia spp. seem to be the dose-limiting nematode parasite for these compounds. While the reason for this differential susceptibility among different nematodes remains unknown, the evaluation of ML concentrations in different sections of the gastrointestinal tract provided some useful information. High MLs concentrations were detected in both abomasal and small intestinal mucosal tissues up to 48–58 days postsubcutaneous treatment to cattle, which accounted for the large AUC values observed in both target sites. These concentration profiles obtained in the mucosal tissue were greater than those obtained in abomasal and intestinal fluids. Only low MLs concentrations (below 1 ng/ml) were found in abomasal fluid following its subcutaneous administration to cattle, which is in agreement with the data previously reported for ivermectin (Bogan and McKellar, 1988), where the drug was not detected in abomasal fluid, even when administered at 10 times the therapeutic dose in sheep. The greater MLs concentrations observed in the small intestinal fluid collected distal of the bile duct, compared with the abomasal fluid, may be associated to the biliary excretion of the drug. The higher lipid composition of the gastrointestinal mucosa compared to the fluids, considered to be a more polar medium, may account for the greater moxidectin availability found in the mucosal tissues. The high availability and extended residence of MLs in the abomasal and intestinal mucosal tissues agree with the reported persistence of the anthelmintic effects of the drug against different nematodes. After oral administration of MLs to sheep, high drug concentrations were measured at the GI tissues of parasite location (abomasum and small intestine) during 3–4 days posttreatment. These concentration profiles were significantly higher than those measured in the bloodstream. The highest drug levels were measured at the abomasal and intestinal contents. These high concentrations in the GI contents are significantly higher than those obtained when the MLs were administered by the subcutaneous route to cattle (Lifschitz et al., 1999a, 2000). The drug concentrations measured in the abomasal content for the MLs reflects the amount of drug accumulated within the adult H. contortus. After the administration of MLs by an enteral route (oral, intraruminal), the access of drug to H. contortus was substantially greater than that obtained following subcutaneous administration. This finding may confirm that an advantageous transcuticular pattern of drug uptake may occur for nematodes located at the GI tract, when lipophilic compounds formulated as a drug solution are orally administered, achieving large availability of soluble drug at the GI lumen (Lloberas et al., 2012, 2013). The comparison of ivermectin and moxidectin concentration profiles achieved in plasma, GI target tissues, and H. contortus collected from infected animals (oral treatments) is shown in Figure 41.7. Figure 41.7 Ivermectin and moxidectin concentrations in plasma, gastrointestinal target tissues, and Haemonchus contortus measured at 1 day after their intraruminal administration (0.2 mg/kg) to infected sheep. Source: Data from Lifschitz et al., 2013. Although differences in feeding mechanisms and location in the digestive tract among different nematodes should be considered to understand the overall action of the ML endectocides, the persistence of the broad-spectrum anthelmintic activity of the drug against adult and immature gastrointestinal parasites is facilitated by its pattern of distribution and prolonged residence in the gastrointestinal tract. The described pharmacokinetic differences between moxidectin and ivermectin combined with some subtle mechanistic pharmacodynamic differences (see Section Mechanism of Action: Ecto–Endoparasiticadal Activity), are likely to be responsible for the observed differences in potency, spectrum, and expression of resistance seen between both molecules belonging to different ML families. The overall comparison of the pharmacological properties of moxidectin and ivermectin is shown in Figure 41.8. Figure 41.8 Comparative pharmacological properties for moxidectin and ivermectin. See the text for further explanation. ML endectocides are highly effective in eliminating mites and suckling lice species after subcutaneous and topical administration. The pattern of ivermectin and doramectin disposition in skin tissue showed that high concentrations of both molecules (>27 ng/g) are attained during the first 8 days posttreatment (Lifschitz et al., 2000). The sustained presence of high concentrations of ivermectin and doramectin in skin were reflected in prolonged mean residence times (6.8 and 9.3 days, respectively), which may also account for the efficacy of these drugs against single-host ticks. The duration of effective concentrations of endectocide compounds in plasma may be important in the treatment of tick infestations, since this parasite can accumulate lethal drug concentrations through its feeding activity over a period of several days. Drug uptake and efficacy against arthropod ectoparasites can be markedly influenced by parasite feeding habits. Thus, the lower efficacy of the ML against biting lice can be attributed to the lower exposure of this ectoparasite to body fluids containing drug. Moxidectin concentrations greater than 9 ng/g were detected during the first 8 days posttreatment in the skin of treated cattle (Lifschitz et al., 1999a), with a peak concentration of 84.2 ng/g achieved at day 1 postadministration. Drug concentrations in skin declined gradually with time posttreatment, as shown for other tissues, being detectable up to 58 days (>0.2 ng/g). Interestingly, the ML concentration profiles in the skin (dermis and epidermis) were greater than those found in the hypodermal tissue. Blood vessels in the skin are limited to the dermis, which receives the largest vascular supply. The dermis participates in the exchange of compounds between blood and tissues and as a fat reservoir (Monteiro-Riviere, 1991). The arthropod ectoparasites are exposed to systemic agents during feeding; the nature of their food source, and frequency and duration of feeding can have a marked influence in drug uptake and efficacy (Jackson, 1989). The ML compounds have exceptional potency and broad spectrum of activity against nematodes and arthropods of different animal species. Their lack of efficacy against trematodes and cestodes may be related to the deficiency of ML-specific binding sites in these parasites (Shoop et al., 1995b). As opposed to benzimidazole anthelmintics, the MLs do not possess ovicidal activity. Compounds from both endectocide families share a similar broad spectrum against endo- and ectoparasites; however, some differences in activity against specific parasites may be observed among different ML molecules. A 0.2 mg/kg dose rate is required for avermectin and milbemycin compounds to eliminate their dose-limiting nematode species such as Cooperia spp. and Nematodirus spp., and to achieve the full broad-spectrum antiparasitic claim in ruminants (Shoop et al., 1995a). Extensive and detailed information on the spectrum of ecto–endoparasitic activity and on the preventive and therapeutic uses of the ML compounds in different animal species has been thoroughly reviewed elsewhere (Armour et al., 1980; Campbell, 1989; McKellar and Benchaoui, 1996; Vercruysse and Rew, 2002). Readers are also referred to the eighth edition of this text (Reinemeyer and Courtney, 2001) for additional specific information regarding the MLs spectrum of antiparasitic activity. However, some overall concepts on the recommended use of the ML in different animal species are summarized below. Macrocyclic lactones compounds are available to be administered to ruminants by subcutaneous (cattle, sheep), oral (sheep and goats) (0.2 mg/kg), and topical (cattle) (0.5 mg/kg) formulations. ML are highly effective against immature and adult stages of different gastrointestinal nematode species from ruminants such as Ostertagia spp. (including inhibited EL4 in cattle), Haemonchus spp., Cooperia spp., Nematodirus spp., Trichostrongylus spp., Strongyloides spp., Bunostomum spp., Trichuris spp., Oesophagostomum spp., and Chabertia ovina. MLs also possess high efficacy against lung worms (Dictyocaulus spp.) in sheep and cattle. The MLs are active against the most of the nematode parasites affecting goats. However, the low systemic availability of some ML compounds obtained in goats (Scott et al., 1990; Alvinerie et al., 1999b) has restricted their use to extralabel prescriptions given at higher dose rates (0.3–0.4 mg/kg) for both oral and parenteral preparations. Arthropod parasites controlled by MLs include mites (Sarcoptes bovis, Psoroptes ovis), oestrid larvae (Hypoderma bovis, H. lineatum, Oestrus ovis), and sucking lice (Linognathus vituli, L. pedalis, and Hematopinus eurysternus). Ivermectin, abamectin, and doramectin are highly effective against Dermatobia hominis infestations. While ivermectin and abamectin aid in the control of the screw worm Cochliomya hominivorax, doramectin has demonstrated a distinctive 100% protection for 21 days postinfection in calves (Moya-Borja et al., 1997). The specific distribution of the MLs in the different skin layers and their prolonged residence agree with its pattern of ectoparasiticide activity. The lower efficacy of the ML subcutaneously administered against biting (Damalinia spp.) compared to sucking lice, may be associated with the superficial feeding of these parasites, particularly on sloughed epidermal cells, which may contain low drug concentrations. The pour-on formulations are highly effective to control both sucking and biting lice. MLs are slightly less effective in controlling the sheep ked (Melophagus ovinus). MLs are highly efficacious against cattle ticks (Rhipicephalus microplus). Ticks are exposed to systemic agents as endectocides during feeding; therefore the frequency and duration of feeding may have a marked influence in drug uptake and efficacy. The ML induces either ticks mortality, lower engorgement, or fewer viable eggs. The injectable ivermectin preparation (1%) shows activity against ticks during 7 days posttreatment. Moxidectin and doramectin provide effective control of ticks for 28 days posttreatment, which is extended up to 75 days in the case of the long-acting 3.15% ivermectin formulations. The ML endectocides contribute to the control of Haematobia irritans (horn fly) in cattle. These compounds kill the adult flies and inhibit larval development in cattle dung. Development of the H. irritans fly may be reduced over 4 weeks after treatment with some ML compounds in cattle. The concept of antiparasitic persistence acquired great clinical significance after the discovery and commercial development of the ML endectocide compounds. The intrinsic properties of the ML and/or their pharmacotechnical preparations allow a sustainable persistence of the active drug in the animal body and therefore prevent the establishment of new nematode burdens for several days after treatment. Large variations in the duration of the ML persistence of antiparasitic activity have been reported, particularly in cattle. The high variability is based on the parasites genera involved, the type of formulation used, different host-related factors, and the design of the experimental trials. For instance, the classic 1% ivermectin and abamectin formulations administered subcutaneously to cattle showed a persistent activity against Cooperia spp., Ostertagia spp., and Dictyocaulus
Macrocyclic Lactones: Endectocide Compounds
General Pharmacology: Avermectins and Milbemycins
Mechanism of Action: Ecto–Endoparasiticadal Activity
Pharmacokinetics
Exchange Between Bloodstream and Target Tissues
Hepatic Metabolism: Bile and Intestinal Excretion
Pharmacokinetic–Pharmacodynamic Relationship
Therapeutic Uses: Animal Species-Specific Considerations
Ruminants
Stay updated, free articles. Join our Telegram channel
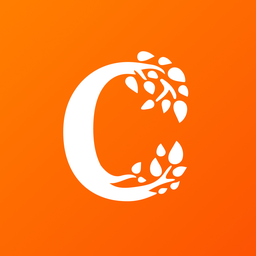
Full access? Get Clinical Tree
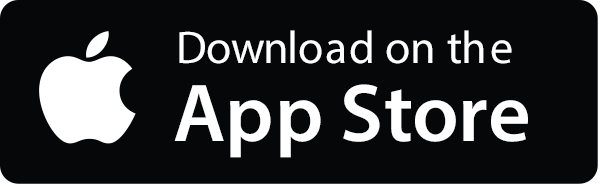
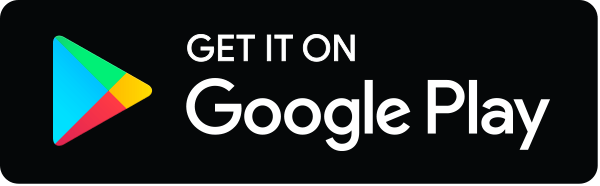