10 Macro and trace elements in equine nutrition
Overview
The elements discussed in this chapter (Table 10-1) are essential for animals as well as for most plants. The differentiation between macro- and trace elements is not linked to their chemical structure but simply reflects their essentiality and average required dietary concentration: ≥100 ppm = macro, <100 ppm = trace element. This approach is very helpful in practice and is linked to content of these elements in animal tissue.
Table 10-1 Macro- and Trace Elements with Nutritional Relevance
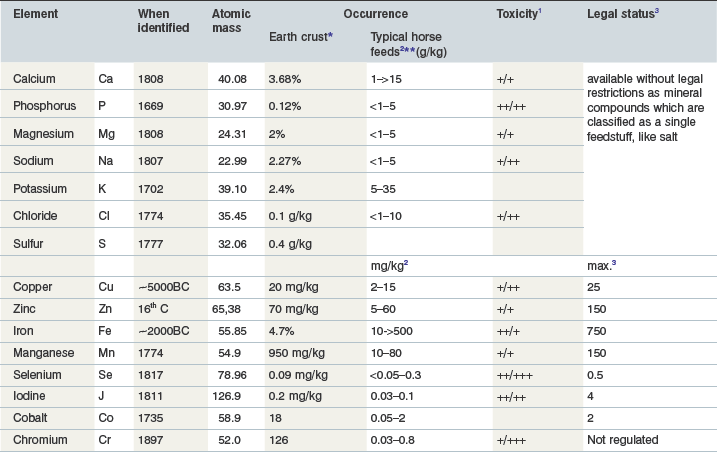
*Merian et al 2004, Anke 2004; **Hohenheim 1976.
1 Indication of the likelihood of toxicity to occur/ how serious or life-threatening such toxicity may be; likelihood: + very rare, experimentally has been produced, ++ may happen, +++ frequently seen; life-threatening if toxic doses are ingested:+ no, ++ possible +++ in most cases; note that the given range for the elements in typical horse feeds are far below toxic levels (see Table 10-4)
3 for the EU; legally defined upper limits for a complete diet and daily ration, respectively, referring to 88% dry matter.
Acute responses: For some elements, effects may be seen within a relatively short time scale when the available amounts do not cover tissue requirements; hypocalcemia is a prominent example. In these circumstances, effects are typically linked to disturbances in the internal balance and distribution of an element even when the intake is not particularly low. Acute responses can also occur if the intake increases to toxic levels (e.g., Se toxicosis), in which cases the high intakes are typically mirrored by abnormal tissue concentrations.
Chronic derangements in mineral intake induce adaptations in the animal; however, even these adaptations can be overridden by critically low or high intakes that may induce nonspecific effects such as growth retardation. Certain tissues become depleted or enriched depending on the intake. The value of ration evaluation to support the development of a clinical diagnosis is commonly underestimated. There might be some difficulties in quantifying feed intake precisely but use of estimates for intake and typical mineral concentrations in the feeds can yield at least sufficient basic information to judge whether one needs further, potentially costly, ration analysis and evaluation or not.
Factors determining requirements
In theory, zero mineral intake, a situation that can occur under experimental conditions, results in a negative mineral balance due to continual mineral excretion, mainly via the feces, urine and skin. The required intake to compensate for these unavoidable losses, defined as endogenous losses, represents the maintenance requirement. Such data, with respect to horses, are available for all of the macrominerals apart from sulfur (S). Data analysis for the balance between intake and retention, based on a broad range of intakes, ideally yields a linear relationship: y = a + bx. The principle is presented in Fig. 10.1. The value for “a” represents the endogenous losses and “b” gives the rate of utilization; both parameters are specific for the individual elements.
The value of “a” ‘(endogenous loss) is influenced by the
1. physiological role of the element
2. dry matter intake (see below)
3. concentration of the mineral in various secretions as part of its role within tissues, e.g., bile secretion
4. capability of an organ to activate conservation mechanisms (e.g. renal reabsorption of sodium).
1. type/ chemical nature of the naturally occurring compound (e.g. CaCO2 vs. Ca-phosphate )
2. digestibility of the feedstuff or supplement containing the element
When considering the requirements for gestation, lactation, growth and exercise, mineral accretion in the respective “products” must be considered, i.e., in the pregnant uterus, in postnatal weight gain, milk, and sweat. As energy and mineral requirements do not change in parallel to the increase in these products, it is not possible to give a certain mineral : energy ratio as a guideline for feeding in practice. Commonly, recommendations are given in g or mg per kg body weight (BW), kg metabolic BW (BW0.75) or per kg feed on a DM basis. However, the accuracy of latter as a target value for rations is low, due to the wide variability in possible DM-intakes (range of 15–>40 g DM/kg BW; see Chapter 3).
• limiting absorption (e.g., Fe) and increasing fecal excretion
• enforced renal excretion (e.g., Na)
Key Points
• Overconsumption of minerals induces an abnormal load in certain tissues and increases the risk of cell damage. This is the case in particular for I and Se.
• When comparing the risk of over-supplementation with Ca or Fe vs. Se or I, it is logical that for rare elements like Se the evolutionary need to establish an “overconsumption management strategy” was quite low (in contrast to Ca or Fe) and therefore the risk of toxicity of such elements through over-supplementation will be higher.
Minerals as essential nutrients
Mineral intake through the ingestion of plants or plant products does not guarantee adequate intake; additional supplementation is often required. The approach to macro vs. trace mineral supplementation is quite different. Macro elements are used fairly freely, provided as oxides, chlorides or sulphates. Some (e.g., CaCl2) can induce mucosal damage and require either high dilution or specific coating to avoid adverse effects. A selection of commonly used mineral compounds is presented in Table 10-2.
Table 10-2 Commonly used Mineral Compounds as Source for Macroelements1
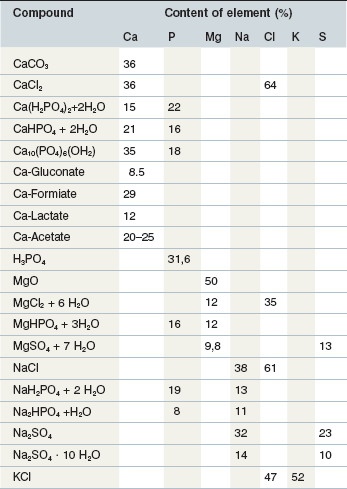
1 Beside the provision of an individual element by the compounds shown above, it is common to use a mineral supplement which combines different elements. A mineral supplement is defined in some countries by the crude ash (CA) content, e.g., Germany min. 400 g CA/kg, and requires specific labeling
Use of feed grade quality compounds helps to ensure safety with respect to contamination by elements that may interfere with utilization (e.g., high Fe) or the uptake of undesirable elements and substances (e.g., Pb and dioxins). The trace elements, partly due to their toxic potential, are regulated by law within the EU and, for certain trace elements (e.g., Se) also in the USA. The EU legal restrictions include the definition of permitted trace element carriers (Table 10-3) as well as the maximum concentration in the diet. Adverse effects are a substantial risk in the case of over-dosage, including negative interaction with the availability of other minerals, tissue accumulation, and the impairment of cellular functions. These factors are considered in defining a safe upper limit (SUL). Current data on SULs are summarized in Table 10-4 (Anke 2004, NRC 2005, Poppenga, 2001).
Table 10-3 Example Compounds Permitted as Carriers for Trace Elements (i.e., Registered for the Provision of Trace Elements which are in Turn Classified as Additives in EU Legislation)
Element | Compound | Content of element (%) |
---|---|---|
Fe | FeCl2 + 4 H2O | 28 |
FeSO4 + 7 H2O | 20 | |
FeII-fumarate | 32 | |
Iodine | KJ | 76 |
NaJ | 68 | |
Ca(JO3) + 6 H2O | 51 | |
Co | CoCl2 + 6 H2O | 25 |
CoSO4 + 7 H2O | 21 | |
Cu | CuCl2 + 2 H2O | 37 |
CuSO4 + 5 H2O | 25 | |
CuCO3 | 58 | |
CuO | 80 | |
Cu(H2H3O2)2 + H2O | 32 | |
Mn | MnCl2 + 4 H2O | 28 |
MnSO4 | 36 | |
MnSO4 + 4 H2O | 25 | |
KMnO4 | 35 | |
Se | Na2SeO3 | 46 |
Na2SeO4 | 42 | |
Zn | ZnCl2 | 48 |
ZnSO4 + 7 H2O | 23 | |
ZnO | 80 | |
ZnCO3 | 52 | |
Zn(C2H3O2)2 +2 H2O | 30 |
Table 10-4 Minimal Requirement Data and Maximum Tolerable Dietary Minerals (data from NRC 2005, Poppenga, 2001, GfE 2013)
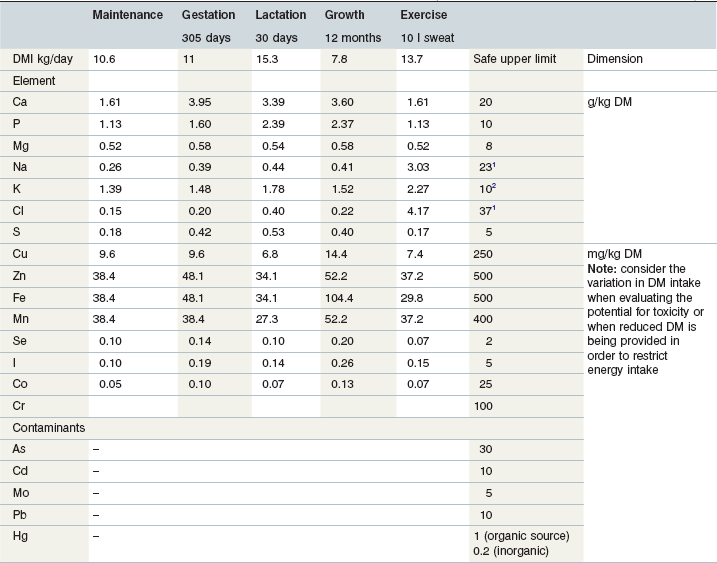
2 >500 mg/kg BW/day may increase water intake and cause diuresis.
Calcium (Ca)
Ca in the pure elemental form is a hard metal (density 1.55 g/cm3); combined with organic or inorganic anions Ca is the most abundant macroelement in the mammalian body. The Ca-content of a newborn foal is close to 20 g/kg BW and decreases to ~15 g/kg BW in adults (Grace et al 1999b, Meyer & Ahlswede 1976). About 99% of total body Ca is found deposited in bone. The biochemistry of Ca in bone ensures two functions: (a) the formation of a tissue with high mechanical load capacity, and (b) buffering of changes in Ca-homeostasis. This fact ensures a high flexibility in Ca turnover via the “feeding” of the mobile Ca pool in blood or muscle by the mobilization of Ca from bone. The import and export of Ca into and out of bone is regulated by calcitonin and parathyroid hormone, respectively.
Major functions
Distribution over tissues
Total body Ca is close to 15 g/kg; the bulk is found in bone. Bone ash (metacarpus, tarsus) contains 385 g Ca (Table 10-5) and 177 g P/kg with no effect of gender or age (Vervuert et al 2010). The fetus has slightly higher Ca content related to the prioritization of bone formation during gestation. Ca accretion is described by an exponential model which reflects that the most intensive bone growth occurs during the last 4 months of gestation.
Ca in bone
As Ca-hydroxyapatite (Ca5(PO4)3(OH)), calcium is an essential contributor to the physical nature of bone and teeth. The high apatite formation and inclusion of Ca in enamel (~38% Ca (Kodaka et al 1991)) produces the highest known mechanical resistant material in the equine body, able to cope with the enormous masticatory forces of >300 N (Huthmann et al 2009). With respect to the radius and tibia, the cortical hydroxyapatite density (commonly taken as bone mineral density, BMD) is 1175 mg/cm3 (Fürst et al 2008). This corresponds to ~470 mg Ca and 87 mg P/cm3. Although there is a strong positive age effect on mechanical strength, at least in metacarpal and metatarsal bones (Glade et al 1986), it seems that the BMD is less sensitive to age (Fürst et al 2008). Bone is formed by collagen fibers (type I, 90% of total protein) and non-collagenous proteins. Spindle- or plate shaped crystals of hydroxyapatite (Ca10[PO4]6[OH]2) are found on the collagen fibers and in the ground substance. The mechanisms of mineralization are not fully understood. The ground substance is composed of glycoproteins plus proteoglycans. These highly anionic complexes have a high ion-binding capacity and are thought to play an important role in the calcification process and in the fixation of hydroxyapatite crystals to the collagen fibers. Osteocalcin, a non-collagenous protein which is synthesized by the osteoblasts, is thought to affect the growth or maturation of Ca2+-phosphate incorporating structures. However, Mg also plays an important role in mineralization as it has been shown to bind to the surface of hydroxyapatite crystals and seems to compete with Ca for the same absorption site (Rude et al 2003, 2009). In addition, studies in laboratory animals have shown negative effects of excessive Mg intake on crystal size and structure as well. In summary, low and high Mg intake in horses has the potential to interact with Ca in the “building of proper hydroxyapatite crystals”.
As a consequence of the biology of bone, Ca and P constitute a mobile component as they can be mobilized from bone on demand in case of a mismatch between intake and the need to maintain homeostasis. This means that the physical properties of bones essentially change during gestation and lactation (Glade 1993, Gruber & Stover 1994). Regardless of Ca intake, a major impact on equine bone quality and bone mineral content is caused by exercise. Stabling horses induces a reduction in BMD whereas natural exercise on pasture as well as short term training bouts induce an increase in BMD, which should result in a corresponding response in bone Ca (Bell et al 2001, Hiney et al 2004, Porr et al 1998). It is evident that the positive impact of natural exercise is underestimated in practice.
Key Points
• ~99% of Ca is deposited in bone and is key in supporting the mechanical property of bone
• Ca in bone can be rapidly mobilized to maintain blood Ca2+ concentrations
• There is no benefit on the mechanical properties of bone when the Ca supply in the diet exceeds requirements
• Immobility/stabling of a horse results in a loss of minerals from the bone – regardless of being provided an adequate Ca intake – and such tissue responses cannot be fully overcome by increasing the mineral supply. The stimulus of physical activity (load bearing exercise) is also required
Other functions
In addition to its contribution to ossification, Ca is involved in blood clotting, muscle contraction, enzyme activities related to energy metabolism, and digestive processes as well as the transmission of nerve signals ((Wijnberg et al 2002a, b, c).
The second highest ranked tissue for Ca content is muscle where the Ca2+ is essentially linked to the contractility of muscle fiber. Inside the muscle cell, Ca shows the lowest concentration, in comparison to other ions, being in the range of 4–19 mmol (160–462 mg)/kg dry matter (DM) (Gottlieb-Vedi et al 1996). Release of Ca from the sarcoplasm and reuptake drive muscle contraction and relaxation (with an impressive exchange rate of ~200 nmol/g muscle). The energy used for maintaining the Ca2+ gradient accounts for ~30–50% of total heat production within the muscle (Hasselbach & Oetliker 1983, Kobayashi & Sugi 1980). The Ca2+-ATPase plays an important role in this aspect of muscle function (Hasselbach 1998); the Ca2+-ATPase varies between 20–29 nmol/kg wet weight in gluteus medius and semitendinosus muscles. There is no influence of age but concentrations are higher in pastured animals in comparison to exercised or stabled horses (Suwannachot et al 2003). The fact that the concentration of ATPase in masseter muscle is only ~20% of the above level may indicate that there is a variable distribution of Ca2+ , accompanied with differences in ATPase activity, according to muscle fiber type.
Ca requirements
Maintenance
The maintenance requirement (see Table 10-5) is related to body weight. In contrast to ruminants there is no effect of DM intake on endogenous losses and thereby on requirements.
Growth
It is evident that any type of growth results in a remarkable increase in requirement.
The Ca concentration in the conceptus increases over time because intrauterine growth shows a preference for connective tissue development in the last 4 months of gestation in order to make the newborn ready for standing and flight-fright responses. The postnatal growth curve (see Chapter 12) determines the development of Ca requirements in the growing foal. Postnatally, in relation to bone, there is a preferred growth of soft tissue and therefore the proportion of Ca in the mature body mass is lower than in a newborn. Therefore, the product “postnatal daily gain” has a lower Ca content vs. preterm gain.
Lactation
On average, Ca in mare’s milk is ~1740 mg/kg but there is a continuous decrease in concentration throughout lactation. The Ca excretion via milk therefore varies according to the day of lactation and milk yield. In fact, the most demanding performance with respect to Ca requirements is milk production (see Fig. 10.2). Although milk yield in mares is difficult to define, a current model based on measured milk volumes and energy requirements of the foal suggests that the maximum mineral excretion via milk occurs within the first 40 days of lactation. Taking into consideration the time dependent drop in mineral concentrations (Ca, P, Mg) in mares’ milk, this results in a nonlinear description of lactogenic Ca export (Fig. 10.2).
Ca balance
Ca intake
The contribution of roughage to total Ca intake is closely linked to the botanical composition of the ration. Alfalfa, clover or herbs like dandelion contain the highest Ca concentrations. Where such plants contribute to the plant community on a pasture, overall content will be >6 g Ca/kg DM. This provides Ca above the requirements for maintenance or exercise if the daily intake of such a pasture is ~20 g DM/kg BW. Typical grass hay provides on average around 4–5 g Ca/kg DM, but it is important to recognize that there is a wide variation in the Ca content of grass and conserved forages; a high percentage of grass forages are below this average value (Fig. 10.3) especially in the UK. This is particularly relevant to the management of Ca nutrition in lactating and growing animals. Cereals in particular are low in Ca (oats > rye & barley & wheat > corn) (Table 10-6). By-products vary between 2 (wheat bran) and >15 g Ca/kg DM (citrus pulp). Cereal straw contains ~1.8 g Ca/kg DM without major differences depending on the source. At DMIs of 80 and 130 g kg0.75 BW for maintenance and lactation, respectively, a Ca intake concentration of 5 g/kg DM results in a Ca supply which would provide ~2 fold and ~1.1-fold of recommended requirements.
The use of mineral supplements is very common in practice, although in most circumstances the feeding of a Ca-containing supplement in addition to average pasture or hay will create a Ca intake that exceeds the requirement (Fig. 10.4).
Key Points
• The presence of significant amount of legumes or certain herbal species (e.g., dandelion) in pasture or hay typically results in >6 g Ca/kg DM. This level of intake covers Ca needs in most circumstances providing that DMI is in the normal physiological range
• High grain rations are likely to provide a low intake of Ca (but high P) if not supplemented
• Typically grass or preserved grass forages may be low in overall Ca content and will require specific countermeasures, such as supplementation, especially for pregnant and lactating mares as well as growing animals
Ca secretion and absorption
Secretion
Saliva (see Table 10-9) contains 157 g/l Ca (Coenen 1985a); assuming 6 liters of saliva production per kg DM of roughage (Meyer et al 1986); this would result in Ca secretion of about 9.4 g/day for a horse consuming 10 kg DM of hay. There is only negligible Ca secretion in the stomach (see Table 10-7). However, the acidic environment may have an important role with respect to Ca availability. The Ca compounds that are of nutritive value (not Ca-oxalates) either from plant tissue or from added minerals are readily soluble in acids. As in other animals, the acidic gastric environment leads to dissociation of Ca, forming Ca2+ ions. This gastric process has two functions: (a) optimizing the solubility of Ca and thereby its absorption, and (b) modulation of gastric acid secretion via the interaction of Ca2+ with Ca-sensing receptors (Ca-sR) and consequent increase in gastrin secretion and gastric acid output. There is some absorption of Ca from the stomach but the majority is absorbed in the small intestine (Schryver et al 1970a, b).
Table 10-7 Estimated Volumes and Mineral Concentrations in Equine Gastrointestinal Secretions (Meyer & Coenen 2002)
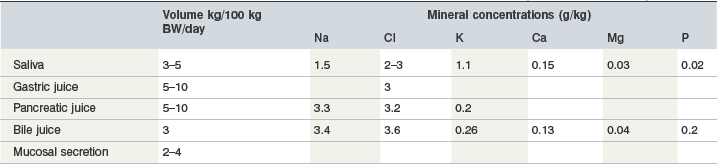
The chronic administration of acid-suppressor drugs like omeprazole has been proposed to decrease Ca absorption in humans (Insogna 2009). Although there are no equine data about this interaction it should be taken as a valid hypothesis that the intensive use of antiacidic drugs will have an impact on Ca absorption. In addition, with respect to the Ca-CasR-gastrin axis, careful evaluation prior to the use of Ca containing mineral supplements is required in order to avoid requirement exceeding intake and vice versa (Shulkes et al 2006).
Ca is secreted in pancreatic juice and bile; the volumes and mineral concentrations are summarized in Table 10-7. Pancreatic Ca secretion is independent of Ca intake but is linked to DM intake (Zebrowska et al 1983). Calcium plays a role in fluid and electrolyte transfer during bile formation. The sensitivity of this function to variations in Ca intake is not known. Cholelithiasis occurs in horses but currently there are no data showing an impact of Ca intake or Ca turnover on this condition.
Absorption
Calcium absorption in the small intestine is driven by a Ca2+ concentration gradient in the lumen and occurs by both trans- and paracellular routes. Foals have higher capacity for Ca absorption but there is no further effect of aging on absorptive capacity. As in other species, binding of Ca2+ to a Ca-binding protein (Calbindin) is the first step in transport (Fullmer & Wassermann 1972). The expression of this protein has been identified in equine placenta, kidney and the intestinal tract, with highest expression in the duodenum (Rourke et al 2010, Wooding et al 2000). Calbindin expression is probably not regulated by vitamin D as Ca uptake in the horse is thought to be independent of vitamin D (Rourke et al 2010). However, recently published studies showed vitamin D receptors throughout the equine gut and an active transmucosal Ca-transport (Sprekeler et al 2011).
Prececal Ca absorption averages ~60–67% of intake (Meyer et al 1982) whereas total tract absorption varies from -5% to 50%, a lower and wider range when compared to small intestinal absorption. This is because the secretion of Ca into the hindgut is related to the amount of fiber in the diet, which obviously varies between 10–40 mg/kg BW/day (Meyer et al 1982, Schryver et al 1970b).
In contrast to ruminants, there is only a weak adaptation in Ca absorption rate in response to dietary intake. A literature survey (Kienzle & Burger 2012 in press) defined a linear relationship between Ca intake (x) and fecal excretion (y) by the following equation: y = 32.11 + 0.54x; r = 0.79, n = 103; intake and excretion in mg/kg BW0.75. From these data, it can be concluded that true digestibility of Ca is ~46%. The Ca : P ratio has no impact on Ca absorption but other factors may reduce Ca absorption. For example, assisted enteral (tube) feeding decreases Ca absorption by ~50% perhaps due to increase rate of intestinal transit (Coenen 1986). Excessive Ca intake (>4-fold of requirement) also reduces the absorption rate (Kienzle & Burger 2012). Feeding fat to horses may negatively affect Ca availability via the formation of Ca salts from unsaturated fatty acids; however, no negative effect of oil feeding on preileal Ca absorption was observed in fistulated animals (Meyer et al 1997). Ca-oxalate is the only naturally occurring Ca compound in feedstuffs that has markedly reduced digestibility. Oxalic acid at >1 % of dietary DM depresses Ca absorption to <10% of intake (Walthall & McKenzie 1976, Swartzman et al 1978, Blaney et al 1981, Hintz et al 1984, Cymbaluk & Christensen 1986, Teleb 1984). Phytic acid, a member of the phosphoinositol family, also forms Ca complexes and limits absorption. Cereals like barley and oats contain up to ~8 g phytate and ~11 g total inositol phosphates per kg DM; these form complexes with P, Ca, Fe, and Zn (Brooks & Lampi 2001). The addition of phytase to the diet improves Ca absorption (van Doorn et al 2004). However, although cereals are high in phytate in typical rations they provide only a minor contribution to total Ca supply. However, the contribution of Ca from the cereal component of the diet can be assumed to be ~10% of the original Ca content. There is no routinely used analytical method for assessment of phytate content.
Ca-excretion
Fecal Ca excretion is linearly related to intake (Fig. 10.5) indicating that absorptive processes do not adapt to differences in Ca intake. Aging also does not appear to affect net absorption (Elzinga, Geor & Harris, personal communication 2011; net absorption, % of intake in mature vs. aged horses, 44 vs. 47). As there is no intestinal adaptation to counteract high Ca intake, the renal route becomes very important with respect to the elimination of Ca when the absorbed amount exceeds requirements and cannot be stored. As such, the kidney acts as a safety relief valve. Effectively, the Ca concentration in urine reflects intake (although sedimentation within the bladder can influence the content in voided sample) and can increase to levels which can provoke precipitation or even stone formation. Urolithiasis in the equine is mostly associated with the formation of insoluble Ca-compounds (Diaz-Espineira et al 1997, Duesterdieck-Zellmer 2007, Laverty et al 1992) as in other hindgut fermenters like manatees and rabbits (Kamphues 1991, Keller et al 2008). See Chapter 36 for more detail on urolithiasis.
A further veterinary aspect is the effect of glucocorticoid treatment in horses. In a dose dependent manner, horses develop negative Ca balance within few days of glucocorticoid treatment due to elevated fecal and renal losses (Glade et al 1982). Glucocorticoids have also been suggested to reduce the activity of the Ca-binding protein in intestinal mucosa (Glade et al 1982).
Regulation of Ca homeostasis
Blood Ca content represents the bound and ionized (Ca2+) fractions. There is no distinct postprandial change in either total Ca or Ca2+ concentration despite the rapid flow of Ca into the extracellular fluid due to highly effective homeostatic mechanisms. In response to an increase in circulatory Ca concentrations, the hormone calcitonin (CT) is immediately secreted (Rourke et al 2009) and subsequently interacts with receptors in the gut, kidney and bone, tissues that can act as a Ca sink (Johnston et al 1989, Rourke et al 2009). The CT response is rapid but brief (Johnston et al 1989, Rourke et al 2009). A decrease in circulating Ca stimulates secretion of parathyroid hormone (PTH); the Ca2+ set point (the Ca2+ concentration at which PTH reaches 50% of its maximum concentration in response to hypocalcemia) for horses is 1.37 mmol/l (Toribio et al 2003) and is higher in comparison to other species. This indicates that feed back signals, mediated by increasing Ca2+ have a delayed response with respect to the reduction of PTH secretion in comparison to other species. The action of PTH on bone as well as the fractionation of Ca in blood (47.4% bound to protein, 48.5% ionized, 4.1% complexed Ca; Lopez et al 2006) restricts our ability to understand Ca homeostasis simply through analysis of blood total Ca concentrations.
Vitamin D supplementation can induce an increase in blood Ca, which suggests that there is a link between 1,25 dihydroxycalciferol and Ca homeostasis. The exclusion of vitamin D from the diet coupled with depression of sunlight-dependent activation of this vitamin resulted in bone abnormalities in horses (El Shorafa et al 1979). On the other hand, vitamin D precursors and vitamin D activity itself are very low in horses (Breidenbach et al 1998, Harmeyer & Schlumbohm 2004). Under physiological conditions, vitamin D intake has minimal effect on Ca homeostasis.
Consequences of failures in calcium intake or deranged homeostasis
There is no significant effect of Ca intake on Ca homeostasis (total vs. ionized Ca) in horses in exercise training (Vervuert et al 2002). Reference values for Ca2+ are lower for lactating mares and foals in comparison to adults at maintenance (Berlin & Aroch 2009). At low Ca-intakes, PTH mobilizes Ca from bone in order to maintain circulating Ca2+ concentration within a narrow range.
A low Ca intake may predispose to tetany, although this condition is rare in horses when compared to dairy cows (Baird 1971, Meijer 1982). Calcium absorption can be impaired by oxalic acid which complexes Ca within the GIT and reduces absorption (Laan et al 2000, Swartzman et al 1978, James & Butcher 1972). A diet providing 1% oxalic acid induced negative Ca balance (Swartzman et al 1978). Rumex species (Rumex obtusifolius, R. crispius) can contain up to ~10% DM oxalic acid (Panciera et al 1990) and this may be a relevant factor in horses on poorly managed pastures.
Under practical conditions, an imbalanced mineral intake is more likely to have an impact on Ca homeostasis than overt deficiency. A relative Ca deficiency can result from high P intake or a low Ca : P ratio. Primary and secondary hyperparathyroidism are the result of a persistent catabolic stimulus of the Ca-sensing system affecting bone. Even the very early reports of these conditions draw attention to the compensatory enlargement of bones (Brevard 1824, Hornbrook 1826). “Big head disease” or Millers disease still occurs even though we have the knowledge on Ca requirements that should prevent this condition (Luthersson et al 2005, Stewart et al 2010).
Defects in Ca-homeostasis are important for some conditions. A short-term shortage in mobile Ca2+ in body fluids can be the result of increased Ca export through milk. Tetanic dysfunction of the muscle in lactating mares can occur towards the peak of lactation (Montgomerie et al 1929, Richardson et al 1991). In the first weeks of lactation, milk Ca is ~1 g/l and is responsible for high losses of Ca from the mare (see Fig. 10.4). The above conditions can be treated or prevented by appropriately adjusting Ca (and P) intake to the increased requirements.
An internal shift in Ca balance via trapping of Ca2+ can influence Ca homeostasis. Hypocalcemia (low Ca2+) occurs during exercise due to the binding of Ca2+ to protein and/or lactate (Vervuert et al 2002). This can result in conditions such as synchronous diaphragmatic flutter (Carlson & Mansmann 1974, Mansmann et al 1974). The exercise induced drop in Ca2+ occurs despite an increase in PTH during physical activity. A two- or threefold increase in Ca intake in comparison to recommendations mitigates the Ca2+ nadir during exercise but cannot maintain normocalcemia (Vervuert et al 2006). Decreased ionized Ca has been observed in horses with severe systemic illness (e.g., colic, endotoxemia, sepsis; Garcia-Lopez et al 2001, Delesalle et al 2005, Toribio et al 2001, 2005, 2007, Hurcombe et al 2009). Moreover, persistence of low ionized Ca was associated with poor outcome during treatment of critically ill horses (Delesalle et al 2005, Hurcombe et al 2009). In other species, some inflammatory cytokines have been shown to decrease PTH secretion, and it is possible that a similar mechanism accounts for low Ca2+ during inflammatory events in horses.
Phosphorus (P)
The total body P content is around 8.6 g/kg (Table 10-8). Similar to Ca, the bulk of body P is in bone (80.8%). Bone ash is about 150–200 g P per kg (Vervuert et al 2010). The P content of bone is age insensitive. Age-related differences in bone composition mostly result from differences in the fat content. Muscle tissue is the second highest ranked tissue with respect to P partitioning in the body but has similar content per gram of tissue to the liver.
Table 10-8 Key Data on Phosphorus in the Equine Body and Requirements
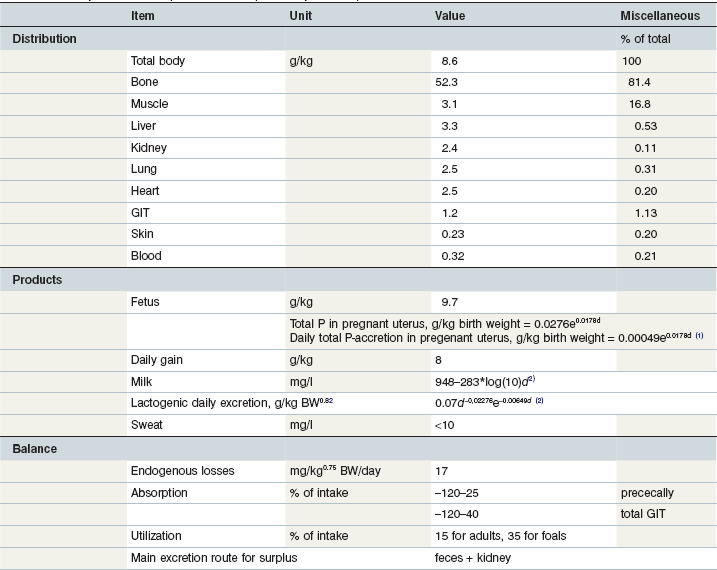
1day of gestation; 2day of lactation.
Coenen et al 2010; Coenen & Vervuert 2003; Grace et al 1999a, b; McCutcheon & Geor 1998a; McCutcheon et al 1995b; Meyer 1990, 1987; Meyer & Ahlswede 1976; Kienzle & Burger 2012; GfE 2011.
Major functions
Phosphorus is required for myriad body functions. For example, P is a component of bone hydroxyapatite and is therefore essential for bone strength. In addition, P is a key constituent of ATP and in the formation of RNA/DNA. Non-osseous body mass shows an inverse Ca : P ratio (e.g., 0.01 in liver and muscle, 0.05 in gut tissue, (Grace et al 1999b). The P concentration inside muscle cells is quite high at 282 mmol/kg DM (in comparison to Ca at 8 mmol/kg DM; Gottlieb-Vedi et al 1996), as would be expected due to the presence of several intracellular P-containing compounds such as enzymes. P is also essential for microbial digestion; low P availability depresses ruminal microbial N-synthesis and digestibility of organic matter and it has been suggested that 75–100 mg P per liter rumen fluid is required to maintain maximum degradative and synthetic microbial activities (Durand et al 1983, Komisarczuk et al 1987a, b, Breves & Schröder 1991). The P concentration in the ileal chyme of the horse is ~0.65 g/kg (Meyer et al 1982) and therefore should provide sufficient amounts for the microbial community. In horses there is substantial P absorption in the hindgut, consistent with other hindgut fermenter species. Hindgut microbes require P but under practical conditions there is no evidence that P can be a limiting resource for large intestinal fermentation.
Requirements
Endogenous losses and other factors important in determining P requirements are summarized in Table 10-8.
Maintenance
The relationship between P intake (x) and fecal losses (y) for adult horses is: y = 7.18 + 0.85x (r = 0.95; n = 265; data in mg/kg BW0.75 per day; Kienzle & Burger 2012). The reported balance data show some differences in the true digestibility related to age (adult 15% vs. young horses 39%).
Pregnancy, lactation, and growth
Lactation increases the P requirement in comparison to maintenance by ~2.8-fold, gestation by ~2.2-fold and growth by ~1.7-fold. The P expenses for lactation vary according to the stage of lactation, described by: P mg/kg milk = 948 – 283 × log day of lactation (for P excretion by milk, see Fig. 10.4) (Coenen et al 2010). The exponential intrauterine growth defines the P budget of the fetus: P g/kg birth weight = 0.0276e0.0178d (d = day of gestation). A constant P concentration is taken for calculating postpartum growth-related requirements. The Ca : P ratio in mare’s milk is clearly below 2 : 1 (see Tables 10-7 and 10-8), which fits with the intensive growth in soft tissues and the delayed gain in bone mass of foals.
Exercise
The enrichment of muscles by mitochondria and other structures bearing P in response to exercise training is accompanied by retention of P in muscle cells (Guy & Snow 1977, Hoppeler 1990, Tyler 1998) and other tissues such as the liver. Regardless of the essential role of P for tissue functions associated with exercise, exercise per se does not appear to be linked to an increased requirement for P. However, young horses entering training need a higher P intake in comparison to maintenance due to tissue growth (bone and muscle). For horses up to the age of 4 years in exercise, the P requirement for yearlings is a reasonable guideline for P supply even if we cannot precisely define the fate of this additional supply.
P secretion and absorption
Secretion
Phosphorus is a constituent of several secretions in the small intestine. This results in a net secretion of P prececally, the extent of which is dependent on the level of roughage intake (Teleb 1984), which increases intestinal secretions. Saliva provides ~20 mg P/l to the preileal P-flux into the gut (Table 10-9). The consumption of 1 kg hay DM is associated with ~6 liters of saliva, which in turn results in saliva-associated P secretion into the gut of 120 mg. Negligible amounts are added by the gastric secretions but the contributions by the pancreatic juice and other secretions in the upper part of small intestine are substantial. Bile secretions are a further source of P (200 mg/l; Meyer 1992). The hindgut also has the capacity for P secretion as well as absorption (Schryver et al 1972); these processes are independent of the dietary Ca : P ratio. However, P absorption in the hindgut determines external P balance (Schryver et al 1972).
Table 10-9 Biochemical Data on Equine Saliva (Coenen 1985b, Eckersall 1984)
Constituent | Unit | Value |
---|---|---|
N | mg/l | 440–585 |
glucose | mmol/l | 34 |
Ca | mg/l | 157–465 |
P | mg/l | 20–54 |
Mg | mg/l | 33–50 |
Na | mg/l | 557–1541 |
K | mg/l | 587–711 |
Cl | mg/l | 780–2130 |
Cu | µg/l | 185 |
Zn | µg/l | 773 |
Fe | µg/l | 3426 |
Absorption
Prececal absorption is highly negative for rations high in roughage (hay and straw, 24.3 and 9.2 mg P intake per kg BW per day → prececal absorption −120 and −35% of intake) but can result in a gain of ~25% of the intake when concentrates are fed (Meyer et al 1982). Consequently, total tract absorption is highly variable depending on intake and the fiber : concentrate ratio.
In Thoroughbreds with intake of 21–22 g P/day, daily P passage through the lower small intestine and upper large intestine were, respectively, ~40–60 g and ~83 g (Matsui et al 1999). A benefit of the absorption in the hindgut is that horses are able to utilize phytate phosphorus, which is made available due to microbial breakdown (Matsui et al 1999). Variation in P absorption is dependent on P intake, source and the type of ration and therefore results in an unpredictable renal excretion with regard to modelling nutrient fluxes. Low renal P excretion can be linked to low intake but also to a ration high in roughage (fiber related elevation in ileocecal P flow and fecal P-excretion). A high renal output of P may reflect different conditions: (a) high concentrate diet that provides the bulk of P intake, and (b) the liberation of P from bone in the case of Ca shortage.
Consequences of failures in P intake or deranged homeostasis
Bone is the target tissue for P deposition and mobilization that is reflected by changes in biochemical markers of bone metabolism such as bone alkaline phosphatase, hydroxyproline or osteocalcin (Lepage et al 2001, Price et al 2001). Bone responds to a dietary P shortage even though blood P concentration does not change, and P supply to the tissues will be maintained as long as bone P can be mobilized. There appears to be a strict priority to maintain P homeostasis at the expense of bone strength.
Key Points
• Prececally, there is net secretion of P into the gastrointestinal tract via saliva, pancreatic juice and other intestinal secretions
• Ileo-cecal P flux supports the microbial community in the hindgut
• The hindgut is the primary site of P absorption, balancing the high prececal secretion
• P intake above requirements results in increased renal P excretion
Magnesium (Mg)
Major functions
About 60% of Mg is stored in the skeleton and 32% in muscle tissue (Grace et al 1999a). In bone, magnesium is associated with the crystalline structure and its incorporation involves the same regulation as for Ca and P. Most of the Mg outside of bone is located in the intracellular space, associated with specific structures such as mitochondria or substrates such as ATP, RNA, or DNA. Most metabolic processes (e.g., oxidative phosphorylation, protein synthesis) inside the cell require Mg, which is one of the most abundant intracellular cations. Dysfunction of the Mg-dependent ATPase attached to myosin is one of the mechanisms underlying clinical signs of hypomagnesemia. Mg deficiency in horses is linked to bone weakness and abnormal mineralization in soft tissues, in particular the aorta (Harrington 1975).
There is an important interaction of Mg with the immune system that is poorly investigated in the horse and therefore the full impact of Mg nutrition on horse health is unknown. In several species, Mg depletion is associated with an increase in proinflammatory cytokines (Tam et al 2003), while increased plasma nitric oxide and lipid peroxidation in cardiac muscle have been shown in Mg-deficient rats (Bussiere et al 2002). An increase in extracellular fluid Mg concentration may be as deleterious as primary deficiency due to augmentation of apoptosis (Black et al 2001). Uterine flushings from dairy cows with abnormal embryos had higher Mg concentrations in comparison to samples from cows with normal embryos (Lamothe & Guay 1970, Wiebold 1988). Additionally, elevated amniotic fluid Mg concentration has been associated with intrauterine growth retardation (Facchinetti et al 1989). Mg is also important in brain function as it is involved in monoamine neurotransmitter synthesis and receptor binding. Mg is essential for the function of N-methyl-D-aspartate-type glutamate receptors that determine memory processing. Mg deficiency therefore should be linked to behavioral consequences. Indeed, studies in laboratory animals show change in fear learning and emotional disruption with Mg deficiency (Bardgett et al 2005). In addition, Mg deficiency has been proposed to play a role in Tourette’s syndrome (Grimaldi 2002). There is consensus that Mg deficits induces neurological symptoms if Mg concentrations in the brain and cerebral-spinal fluid are depressed (Morris 1992). On the other hand, very high doses of Mg (e.g., administered IV) induce anesthetic effects.
Mg depletion in horses also has been associated with brain malfunction (MacKay 2004, Stewart 2011, Stewart et al 2004). However, to date there are no published data from well-designed studies to demonstrate that increased Mg intake improves brain function or ameliorates excitability in horses.
Requirements
Maintenance
Fecal and renal losses for Mg are linearly related to Mg intake (x): fecal losses = 12.17 + 0.54x (r = 0.93, n = 258); renal losses = 11.69 + 0.19x (r = 0.64, n = 128; variables expressed in mg/kg0.75 BW/day1; Kienzle & Burger 2012). Average endogenous losses are 23.9 mg/kg0.75 BW/day and the estimated utilization rate is 46%.
Gestation, lactation, and growth
Gestation and lactation result in substantial increase in Mg requirements (Table 10-10) along with needs for Ca and P. The output of Mg via milk (mg/kg0.82 BW/day = 0.0092d−0.0111e−0.00627d, d = day of lactation (Coenen et al 2010) reflects the time related decline in milk Mg concentration and the change in daily milk volume. A constant Mg concentration in daily gain is used to calculate growth related requirements. The absorption efficiency for suckling foals will be higher than the average figure for adults (see Table 10-9). Currently, the calculated requirement for growth may be overestimated in foals in the first 2 months of life.
Table 10-10 Key Data on Magnesium in the Equine Body and Requirements
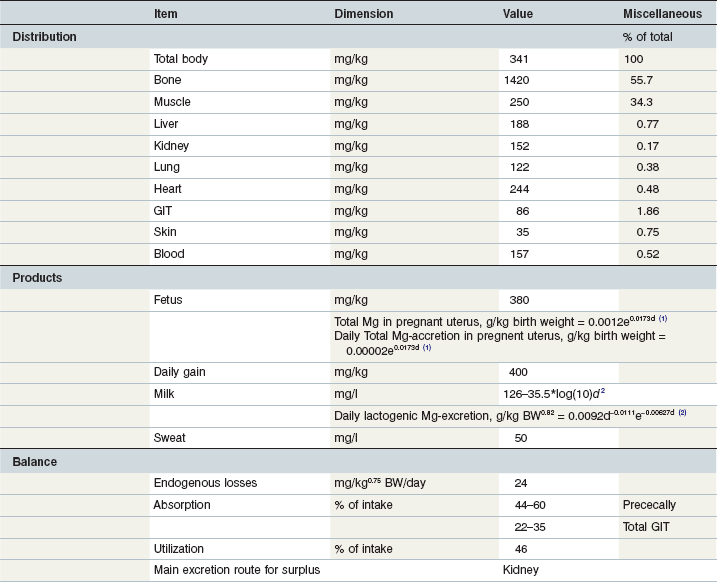
1Day of gestation; 2day of lactation.
(Coenen et al 2010; GfE 2013; Coenen & Vervuert, 2003; Grace et al 1999a, b; McCutcheon & Geor, 1998a; McCutcheon et al 1995b; Meyer 1990, 1987; Meyer & Ahlswede 1976; Kapusniak et al 1988; Kienzle & Burger 2012; GfE 2011).
Mg secretion and absorption
Mg secretion in the upper GIT is small (see composition of saliva and pancreatic juice) with more substantial secretion in the hindgut. The net absorption of Mg over the entire GIT tract varies between 5% and 60% of intake (Hintz & Schryver 1972, Meyer et al 1982). The small intestine is the primary site of absorption (~45–60%; Meyer et al 1982), and therefore under physiological conditions net Mg secretion occurs in the hindgut. However, Mg can be absorbed in the large intestine if the concentration in the chyme is elevated (e.g., by rectal infusion). A linear relationship between Mg intake (x) and fecal excretion over a wide range of intake (fecal Mg mg/kg BW/d = 0.51 + 0.54 + Mg intake, mg/kg BW/day (von Bieberstein 1990)) indicates the independence of absorption from intake. High Mg intake elevates Ca absorption and vice versa (Hintz & Schryver 1973). The surplus ingested Mg is excreted via the urine. The regression equation y = 2.18 + 0.17x (Meyer & Staderman 1990) describes this relationship between Mg intake (x, mg/kg BW/day) and renal output (y, mg/kg BW/day) and has been confirmed in review of literature data (Kienzle & Burger 2012).
Consequences of failures in Mg intake or deranged homeostasis
Total Mg in plasma is between 0.5 and 1.11 mmol/L; ionized Mg varies between 0.38 and 0.63 mmol/l (Berlin & Aroch 2009). In ruminants there is a negative interaction between high dietary protein intake and K plus Mg absorption in the rumen. It is not clear whether the Mg-absorption in the equine hindgut responds to the high ammonia concentration and high K influx. The link of Mg to bone and mitochondria suggests that insufficient Mg supply will affect bone strength and energy metabolism. But the dominant response in all animals to an inadequate Mg intake is poor performance (e.g., growth), increased disease risk due to immune-incompetence and muscle weakness. Animals demonstrate tetanic cramps as the most pronounced clinical sign of hypomagnesemia.
In vitro studies have shown that 35S incorporation into chondrocytes is decreased when they are incubated in Mg-deficient medium (Davenport et al 2001). Mg deficiency therefore might increase risk of orthopedic diseases, particularly in growing horses, but there is no evidence linking Mg nutrition to bone abnormalities. Harrington 1975 reported hypomagnesemia and associated Mg losses from bone when horses were fed a low Mg diet (7–8 mg Mg/kg vs 390 mg Mg/kg as control). In horses with enterocolitis, both hypocalcemia and hypomagnesemia occur in association with low PTH (Toribio et al 2001) suggesting failure of regulatory mechanisms during illness. In healthy animals fed typical diets, blood Mg concentration is an insensitive indicator of Mg intake whereas reduced renal excretion may reflect marginal or deficient Mg nutrition (Stewart 2011, Stewart et al 2004).
Electrolytes (Na, K, Cl)
Cell functions require a well controlled water-based environment, the main characteristics of which are a specific acid–base-balance and osmotic condition. The electrolytes sodium (Na), potassium (K) and chloride (Cl) are not exclusively responsible for ensuring such an environment is present, but they are indispensable. The outstanding position of Na and Cl is represented by the central regulation of salt appetite (Geerling & Loewy 2008).
Major functions
The skeleton contains ~51% of the body’s Na, while K is found mainly in muscle (75%; Lindner 1983, Gürer 1985). Several tissues contain around 10–15% of total body Cl (Coenen 1991). The key data on body distribution and balance are summarized in Tables 10-11–10-13. Movement of the electrolytes Na, K and Cl across membranes are the major driving forces for the distribution of water and have a significant impact on the electrochemical properties of the body’s water compartments. The osmolality of the extracellular water space (ECW) and blood plasma depends mainly on the concentration of these elements. As the most abundant cations and anions, they are major contributors to acid–base balance. Changes in the dietary profile of Na, K and Cl will induce changes in systemic pH and bicarbonate concentrations as well as urinary acidity (Baker et al 1998, 1993, Coenen 1991, Stürmer 2005, Stutz et al 1992, Wall et al 1992). The Na–K pump maintains a large difference in the intra- (K) and extracellular (Na) location of these two cations, and serves other functions regarding the substrate equilibrium in and outside the cell. Further transport functions are linked to Na-symporter systems. For example, iodine transport through the thyroid cell membrane occurs in association with Na. Muscle contraction induces a change in the intracellular/extracellular ratio through liberation of intracellular K and is reflected in an exercise intensity-dependent increase in plasma K concentration. Chloride is the most abundant inorganic anion; Cl homeostasis interacts with bicarbonate retention or excretion in order to maintain systemic acid–base balance. Cl also has a specific role in gastric hydrogen ion secretion (Shulkes et al 2006) and in respiratory CO2 elimination. Na, K and Cl appear in most secretions in concentrations close to those in blood. Interestingly, these electrolytes have limited flexibility with respect to internal balance as there is no tissue which serves as a depot. On the other hand, there is large flexibility in external balance that is managed via renal retention and excretion depending on intake and utilization (i.e. extra output in milk or sweat). Fecal losses do not respond to these factors see Fig. 10.6A). Increased Na excretion by sweat is mirrored by a reduced renal excretion (Coenen & Vervuert 2003). By this simple strategy, Na homeostasis is maintained over a wide range of intakes and in the face of acute changes in utilization see Fig. 10.6B). This is extremely important in the case of sweat production; the sweat gland is unable to modify sweat Na concentration to any great extent. This is also true for Cl which can reach ~5.5 g/kg sweat and therefore cutaneous Cl losses can add up to ~20% of total body Cl. The actual changes in overall electrolyte homeostasis that occur in the face of such losses are minimal or quite small in comparison to the actual amount lost in the sweat due to the efficiency of renal conservation mechanisms. Fig. 10.6 shows the change in renal Na excretion between rest and exercise. The increase in cutaneous loss (e.g., Cl) is mirrored by a decrease in renal excretion (Fig. 10.7).
Table 10-11 Key Data on Sodium in the Equine Body and Requirements
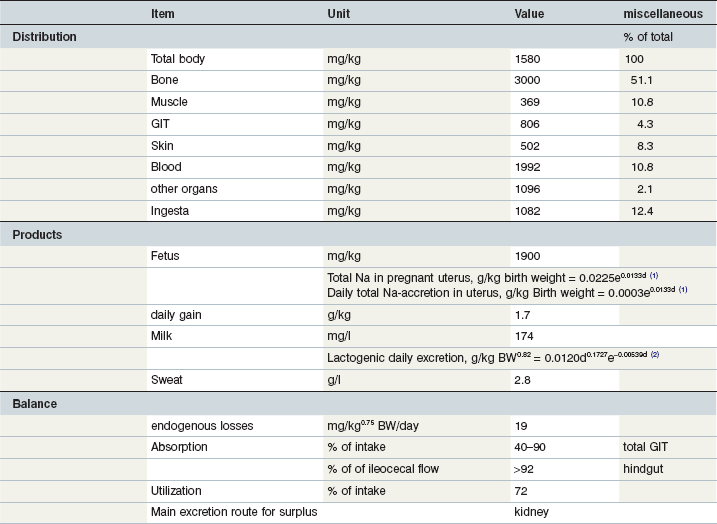
1day of gestation; 2day of lactation.
Coenen et al 2010; GfE 2013; Coenen & Vervuert, 2003; Grace et al 1999a, b; McCutcheon & Geor, 1998a; McCutcheon et al 1995b; Meyer 1990, 1987; Meyer & Ahlswede 1976; Kapusniak et al 1988; Kienzle & Burger, 2012; GfE 2011.
Table 10-12 Key Data on Potassium in the Equine Body and Requirements
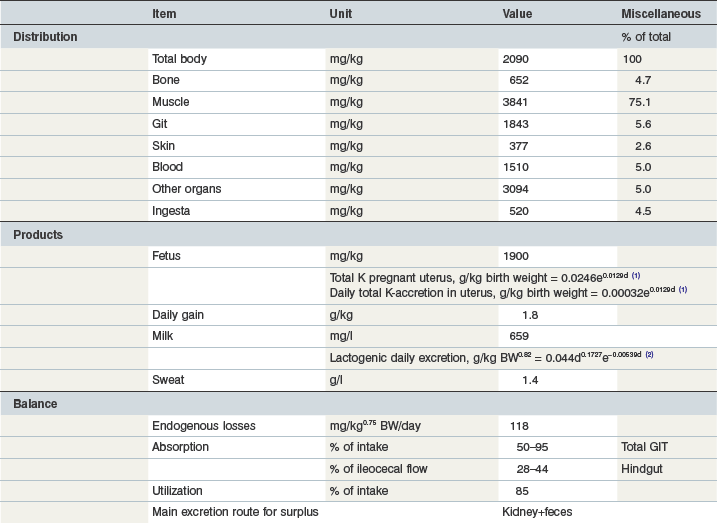
1day of gestation; 2day of lactation.
Coenen et al 2010; GfE 2013; Coenen & Vervuert, 2003; Grace et al 1999a, b; McCutcheon & Geor, 1998a; McCutcheon et al 1995b; Meyer 1990, 1987; Meyer & Ahlswede 1976; Kapusniak et al 1988; Kienzle & Burger, 2012; GfE 2011.
Table 10-13 Key Data on Chloride in the Equine Body and Requirements
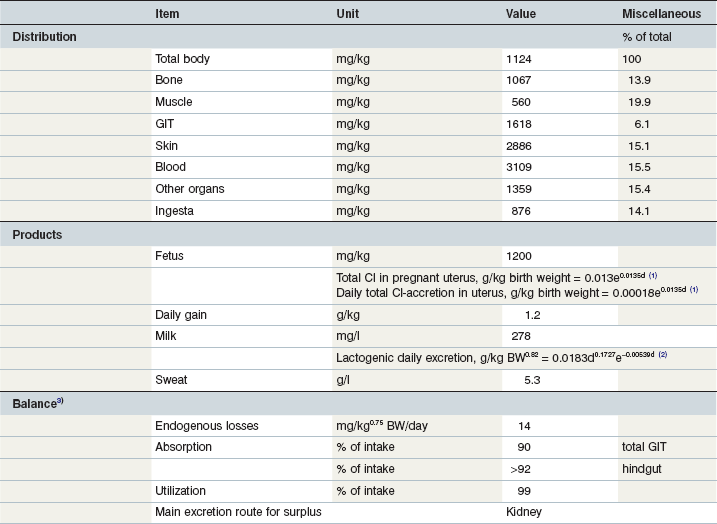
1Day of gestation; 2day of lactation; 3the Cl intake at the level calculated by the tabled data accordingly the factorial approach is rather low and may affect normochloremia and acid–base balance.
Coenen et al 2010; GfE 2013; Coenen & Vervuert, 2003; Grace et al 1999a, b; McCutcheon & Geor, 1998a; McCutcheon et al 1995b; Meyer 1990, 1987; Meyer & Ahlswede 1976; Kapusniak et al 1988; Kienzle & Burger, 2012; GfE 2011.
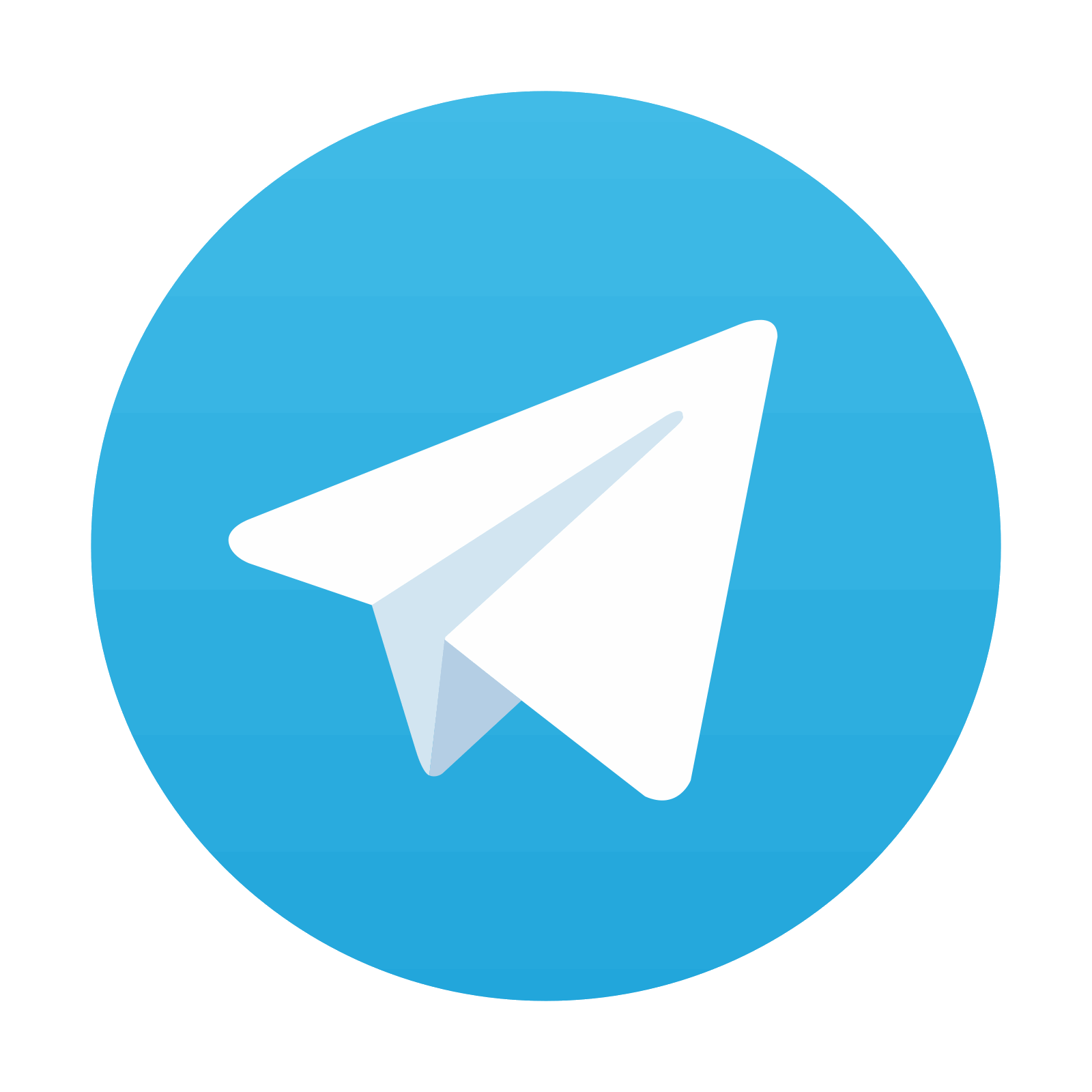
Stay updated, free articles. Join our Telegram channel
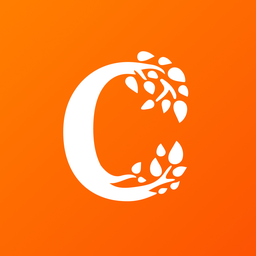
Full access? Get Clinical Tree
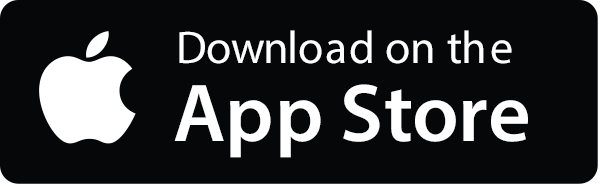
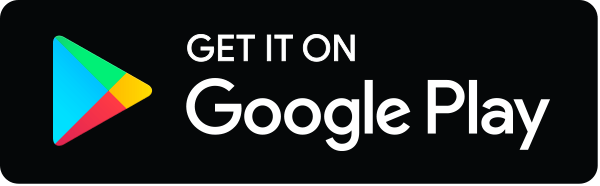