The lasers used in rehabilitation help to modulate cellular functions. This process is known as photobiostimulation and is defined as nonthermal interaction of monochromatic radiation with a target site.1 Although the physiologic interaction of this type of energy application on tissue is still not completely understood, low-energy lasers have been reported to modulate various biologic processes, such as mitochondrial respiration and adenosine triphosphate (ATP) synthesis, to accelerate wound and joint healing, and to promote muscle regeneration.2,3 In addition, acute and chronic pain control has been reported using this type of low-energy photon therapy.4 Treatment of chronic and acute edema, neurologic conditions, and postoperative care are some other popular conditions treated with laser therapy. Basic light sources emit electromagnetic radiation that is visible to the normal eye. Although natural light sources, such as sunlight, are forms of electromagnetic radiation, lasers are artificial sources that emit radiation in the form of a flow of photons (Figure 21-1). The process of light emission begins with activation of electrons in the laser unit, generally either helium-neon (HeNe), gallium-arsenide (GaAs), or gallium-aluminum-arsenide (GaAlAs) to an excited state.5 When the electrons drop from their excited state to their ground state, photons are emitted. Although some photons are absorbed by the laser chamber wall, others stimulate the emission of other photons, and together they travel in the chamber, amplifying this stimulated emission, which results in a chain reaction. Some of these photons are released through a semireflective mirror to form a beam of light. The major difference between laser light and light generated by normal sources is that laser light is monochromatic, coherent, and collimated. Monochromatic means that all light produced by the laser is of one wavelength, and therefore a single color. Sunlight, or white light, may be broken into several different colors of different wavelengths by a prism (Figure 21-2). Laser light has electromagnetic radiation of only one wavelength. Commercial lasers occasionally have two or more different wavelengths within a single unit to achieve different effects, but each component within the unit has a single wavelength and is monochromatic. The coherent properties of light mean that the photons travel in the same phase and direction (Figure 21-3). Laser light is also collimated, which means that there is minimal divergence in the laser beam over a distance (Figure 21-4). Using a monochromatic light source allows the absorption of the light to be targeted to specific wavelength-dependent chromophores, or photon acceptors.5 The properties of coherence and collimation allow the light to be focused precisely on small areas of the body. These properties allow low-level laser light to penetrate the surface of the skin with no heating effect, no damage to the skin, and few or no side effects when properly used. Laser light interacts with tissues in various ways. Light may be reflected, scattered, transmitted, or absorbed. Reflected photons have no clinical effect and may be dangerous to tissues that encounter reflected photons, such as the eyes. In addition to surfaces such as watches, tables, and instruments, the epidermis is responsible for reflecting most of the photons from the skin. To reduce reflection of photons, the laser beam should be directed as nearly to 90 degrees as possible to the skin surface. As photons pass through the tissues, some are scattered. Each photon that is scattered when striking an object reduces the amount of energy that can be directed at the target tissue. Scattering decreases as the wavelength increases because longer wavelengths penetrate deeper into tissues. Transmitted photons pass completely through the tissue without being absorbed. This is rarely a problem in rehabilitation because the tissues are generally thick enough to prevent complete transmission. Finally, photons may be absorbed. Photons are absorbed by chromophores (molecules that absorb certain wavelengths of light) and it is in this manner that laser may affect tissues. The most common chromophores are water, hemoglobin, melanin, cytochrome C system in mitochondria, proteins, and amino acids.6 The relative amounts of chromophores vary among tissues, and the absorption of photons by chromophores varies, in part, on the wavelength. The wavelength of the photons is important in laser therapy. Wavelengths are measured in nanometers (nm) and determine, in part, the biologic effect on tissues. For example, ultraviolet light (100-400 nm) is absorbed primarily by melanin, proteins, and nucleic acid; visible light (400-760 nm) is scattered and absorbed, with the absorption primarily by melanin, hemoglobin, and myoglobin; with near infrared light (760-1400 nm), photons are mainly scattered, but a variety of chromophores absorb these photons, although somewhat weakly; in the far infrared zone (1400-10,000 nm), absorption is almost entirely by water (Figure 21-5).6 Therefore the optimal window to have tissue penetration with less scattering and surface absorption is likely to be in the 600-1200 nm range. The energy density, or radiant exposure, is the energy per surface unit and is typically indicated by joules per cm2, or J/cm2. This term is also clinically called the dose of laser energy and is important in determining and describing treatment protocols. To date, only the dose of laser energy has been shown to have biologic effects as compared with the rate of administering laser energy, or the power of the laser (Box 21-1). Greater doses of laser energy penetrate deeper into tissues (Figure 21-6). The Lambert-Beer law indicates that for homogenous tissues with a constant coefficient of absorption, more photons will reach a given tissue depth with a greater dosage, and the intensity of light passing through tissues decreases exponentially with increasing tissue depth. There is a great deal of difference among lasers related to the power, with those delivering energy at a greater rate generally costing more. Although there is not thought to be an advantage in efficacy regarding low- versus high-power lasers, the dosage (total joules) can be administered in a much shorter time with a high-power laser, therefore reducing labor costs. In addition, high-power lasers are generally administered in a sweeping fashion, whereas lower power lasers are usually held in place until the administration of the dose for a particular spot treatment is complete. The sweeping motion may result in more thorough coverage of the treatment area as compared with the spot treatment of lower power lasers. The sweeping motion also allows the clinician to cover other surrounding areas that may be causing secondary or tertiary pain. For example, when treating canine hip dysplasia to address pain and inflammation, the coxofemoral joint should be treated from all areas—medial, lateral, caudal, and cranial. With the sweeping motions covering the four areas, the laser may affect the surrounding soft tissues that are often painful in clinical canine hip dysplasia. Lasers can emit photons continuously or in a pulsed fashion (Figure 21-7). With continuous wave laser, radiation is emitted at a constant power over the entire treatment time. With pulsed wave laser treatment, impulses may be emitted at varied rates, with an on time when energy is emitted (pulse width or pulse duration [PD]) and an off time when there is no energy emitted. The duty cycle (DC) is the percentage of time that radiation is emitted in relation to the total on-off time. The pulse rate, or frequency (F), is measured in Hertz (Hz). The relationships between these measures may be expressed as: One report reviewed the literature regarding pulsed versus continuous laser application.7 Although the authors concluded that there is some evidence that pulsed laser has different effects than continuous laser, further work is necessary to define optimal treatments for various conditions, and to determine the optimal pulse structures. One theory behind the use of pulsed laser is that with relatively short pulses, the laser could be excited to higher levels as compared with continuous wave laser, in which thermal damage to the tissues might limit the maximum amount of energy that can be applied. With short pulses, the thermal effects on tissues might be reduced, limiting tissue damage. Pulsed GaAs and indium-gallium-arsenide (InGaAs) lasers may allow for deeper tissue penetration without thermal damage, as well as allowing for shorter treatment times. In addition to decreasing the thermal effects on tissues, pulsing of the laser may also have some resonance with certain functions, such as brain waves and opening of cellular ion channels. Pulsing may also have effects on photodissociation of substances, such as nitric oxide (NO) from protein binding sites, allowing multiple episodes of dissociation. Although the actual depth of penetration is probably not that different in pulsed versus continuous wave lasers, for many conditions, depth of penetration is a less important issue, such as with treatment of elbow or stifle arthritis. One author concluded that pulsed lasers (904 nm) were not significantly more effective than continuous-wave lasers (810-830 nm) for treatment of tennis elbow in people.8 Both types were equally effective, but half the energy was needed with pulsed lasers. However, a review of literature comparing continuous wave laser with pulsed laser identified six of nine studies that found pulsed wave to be more effective.7 A clinical study of wound healing and experimental studies of pain management and stroke recovery have suggested that pulsed laser has better results than continuous laser. Another study comparing continuous and pulsed laser found both to be equally effective. Only two of the nine studies reported better results with continuous than pulsed laser, although both treatment methods achieved better results than placebo treatments. Some limitations of the studies in this review were that the same parameters of laser wavelength were not used in some instances, making direct comparisons difficult. One study found a combination of pulsed and continuous laser to be more effective than either alone for stimulating nerve regeneration in a median nerve transection and repair study,9 whereas most studies have shown continuous laser to be more effective than pulsed laser for nerve conduction and regeneration.7 Studies comparing various rates of pulsed laser treatment show no consistent results, with some demonstrating better results with lower frequencies and some with higher frequencies.7 The American National Standards Institute in the United States and the International Electrotechnical Commission have defined classes of lasers based on their ability to damage tissues. In general it is the thermal damage that injures tissues, especially the eyes, and in some cases, skin. Even small amounts of laser light can cause permanent damage to the retina.10 Class 4 lasers have the greatest potential to cause tissue damage and include all lasers with power greater than that of Class 3B lasers (Figure 21-8). Surgical, industrial cutting lasers, and some therapeutic lasers are Class 4 lasers. Surgical lasers typically have power between 30 to 100 W, whereas therapeutic lasers may be 1 to 15 W. Class 4 lasers may burn the skin or cause permanent eye damage as a result of direct, diffuse, or indirect beam viewing, such as might occur with reflection of the beam, even from matte surfaces. Therefore, the therapist must use great care to control the beam path and protective eyewear must be worn by all in the immediate area. The U.S. Food and Drug Administration (FDA) requires all Class 3B and Class 4 lasers in the United States to have safety features, including a key switch, a safety interlock dongle, a power indicator, an aperture shutter, and an emission delay (normally 2-3 seconds). In addition, the U.S. Occupational Safety and Health Administration requires the use of adequate eye protection when eye exposure may occur while operating lasers in these classes. The wavelength of the laser is important because it determines, in part, the laser’s effect. Longer wavelengths are more resistant to scattering than shorter ones. Therefore, GaAs and GaAlAs lasers penetrate more effectively (direct effect up to 2 cm, indirect effect up to 5 cm) than HeNe lasers (direct effect up to 0.5 cm, indirect effect up to 1 cm) because there is less absorption or scattering in the epidermis and dermis. Light waves in the near infrared ranges penetrate the deepest of all light waves in the visible spectrum (Table 21-1). Although this spectrum of light is not visible, commercial lasers should have an LED that allows the therapist to see where the laser light is aimed. Table 21-1 Wavelengths of Various Components of the Electromagnetic Spectrum Lasers can only have an effect if they stimulate cells, and the depth of laser penetration is important to determine if cells at a particular depth may be stimulated. Very few studies have measured the depth of laser penetration through skin, and these studies have been done primarily on human skin samples. Readers should use caution in interpreting the results of these studies and extrapolating them to dogs because of inherent differences in skin thickness, skin composition, cutaneous blood supply, skin color, and thicker hair coats. Lasers with shorter wavelengths are most effective for surface-level conditions because they do not penetrate to deep tissues or large joint capsules. Wound care and wound healing should benefit from lasers with shorter wavelengths. Light that is not absorbed by water, hemoglobin, or melanin is gradually attenuated as it passes through tissues. The level of scattering and absorption is such that HeNe (632.8 nm) laser light loses approximately one third of its intensity during the first 0.5-1 mm of tissue depth.11 The depth (in centimeters) at which the energy of a laser beam is 36% of its original values is termed the first depth of penetration.12 This attenuation of energy is derived by dividing the original value by a constant, 2.78. Subsequent depths of penetration may be determined by dividing by 2.78 again, so that the level of energy at the second depth of penetration is 13%. However, because biologic effects may be noted with relatively low energy (0.01 J/cm2), lasers that typically deliver 1 to 4 J/cm2 may penetrate up to 0.5 to 2 cm before the energy level is so low that they have no effect. These factors apply to human skin, and, although there are many similarities between human and animal skin, there are significant differences caused by hair, pigmentation, and tissues. One study evaluated the penetration of ruby laser light (694 nm) in human white skin. Doses of 4.75 J/cm2, 9.24 J/cm2, and 13.41 J/cm2 were administered to the skin.13 (see Figure 21-6). The most likely explanation for the tremendous drop in energy in the first mm is scattering of laser light, which supports the hypothesis that the greatest hindrance to a photon energy beam traversing the skin is from the stratum spinosum of the epidermis, collagen in the dermis, or both. Beyond the depth of the dermis and at the levels of the subcutaneous fat, the energy drop per distance traveled was not as great. The mean maximum depth of penetration was 14.8 mm which appeared to be a function of wavelength and not dosage. The natural chromophore for this wavelength of laser is melanin. Therefore the depth of penetration might be expected to be less in dark-skinned dogs. After passing through the skin, penetration through the muscle becomes easier because muscle has a smaller scattering coefficient.14 In fact, transmission of laser light between 600 and 800 nm is approximately fourfold greater in skeletal muscle than skin.15 Another study with laser of similar wavelengths had similar results. An HeNe laser (632.8 nm, 50 mW power) and a semiconductor laser (675 nm, 21 mW power) were used to measure transmission in human skin and skin with granulation tissue from leg wounds with ulcers.16 In the thickest sample (epidermis, dermis, and subcutaneous tissue, 2 cm thickness), approximately 0.3% of HeNe laser and 2.1% of semiconductor laser light penetrated through all layers. Penetration of laser light was greatly attenuated at 1 to 3 mm of tissue depth. Transmission in granulation tissue was approximately 2.5 times higher than that in normal skin. In another study of depth of penetration, a GaAlAr laser (wavelength 850 nm, 100 mW power) was applied to human abdominal skin samples.17 The intensity of laser radiation was reduced by 66% after being transmitted through a 0.784 mm sample of human abdominal tissue. In this study, most laser radiation was absorbed within the first 1 mm of skin. Additional research is needed in small animals to determine the depth of penetration with different hair coats and skin color. Most studies of laser use in rehabilitation have centered on wound healing and pain management. However, information regarding their efficacy in reducing pain or promoting tissue repair is incomplete.18 Recently, interest has been generated in the United States regarding their use in treating people, and a natural extension has been an interest in treating animals. In evaluating the potential usefulness of laser therapy in rehabilitation, the reader is encouraged to be critical of studies that have been performed, and to evaluate these studies in light of recent advances in laser technology and the application of new information. Until recently, low-energy and therapeutic lasers were not approved for medical treatment in this country. However, as more evidence becomes available, they will likely be increasingly used. Most of the potential responses of cells and tissues to laser energy have been studied in in vitro models. Photons delivered to the cells and tissues trigger biologic changes within the body. Photons are absorbed by chromophores and respiratory chain enzymes (especially cytochrome C oxidase, the terminal enzyme of the mitochondrial respiratory chain) within the mitochondria and at the cell membrane. Copper components of cytochrome C oxidase are photoacceptors.19 Cellular signaling causes a cascade of cellular reactions resulting in things such as NO dissociation from cytochrome C oxidase.19 This, in turn, results in further changes down the respiratory chain. Oxygen production and the formation of proton gradients across the cell and mitochondrial membranes may also occur. The enzyme flavomononucleotide is activated and initiates the production of ATP. Even small changes in ATP levels can change cell metabolism. The role of ATP in cellular energetics is well known, but ATP may also act as a signaling molecule to enhance cell to cell communication.19 ATP may bind with the cell receptor P2X, which opens a channel to allow sodium and calcium to enter cells, resulting in a cascade of intracellular interactions. Increased intracellular calcium positively affects mitochondrial function. ATP may act as a neurotransmitter when released by nerve cells, but may also have other effects when released by other cells, such as bone production and cell proliferation.20 The changes in ATP may, through its effects as a neurotransmitter, explain some of the effects of laser therapy on pain modulation and the effect of acupuncture. The effect of laser treatment on various cells in vitro has been evaluated in several studies.21 HeNe laser (632 nm) with a power of 10 mW and a dose of 0.43 J/cm2 resulted in greater proliferation and differentiation of human osteoblasts in vitro.22 DNA production may also be stimulated. Photons also appear to affect tissues by activating enzymes, which trigger biochemical reactions in the body. Because cellular metabolism and growth are stimulated, lasers have the potential to accelerate tissue repair and cell growth of structures, such as tendons, ligaments, and muscles. Although lower dosages of laser energy appear to stimulate tissues, higher dosages may actually inhibit responses such as tissue healing. The enzyme kinetics may differ between high enzyme/substrate, and low enzyme/substrate conditions.23 A great deal remains unknown regarding the mechanisms of laser light in biologic systems. For example, NO and carbon monoxide inhibit cytochrome C oxidase. The effects of near infrared laser radiation in conjunction with NO on cytochrome C oxidase are unknown.24 Further work is also needed regarding the effects of temperature, pH, exposure times, and frequency of photobiomodulation treatment on cytochrome C oxidase. Laser therapy may also stimulate stem cell proliferation.19 In addition to the direct effects of laser at a particular depth of tissue, indirect effects may also be seen. These cellular and tissue effects are decreased in the deeper tissues, and are catalyzed by the energy absorption in the more superficial tissues. Laser therapy may also have antiinflammatory effects, similar to nonsteroidal antiinflammatory drugs (NSAIDs) and steroids. Studies of laser therapy in cell culture have indicated that inflammation can be reduced by a decrease in prostaglandin E2 (PGE2) and cyclooxygenase-2 (COX-2) concentrations.25,26 This effect has also been demonstrated in an animal model,27 as well as people with Achilles tendinitis.28 Frequently, owners seek alternatives to NSAIDs for their animals, and laser therapy may offer alternative care. Animals that are unable to consume NSAIDs may also benefit from laser therapy as an alternative for inflammation. Laser light stimulates fibroblast development and may affect collagen production to repair tissues. Laser light may also accelerate angiogenesis and increase the formation of new capillaries in damaged tissues, possibly improving the rate of wound healing (Box 21-2). Therefore laser therapy may aid healing of open wounds and burns. There is an increased growth factor response within cells and tissues, which may be related to increased ATP and protein synthesis. Laser light therapy causes vasodilation and also may improve lymphatic drainage. This may result in decreased edema and swelling caused by bruising or inflammation. In cell culture, doses of 0.5 to 16 J/cm2 were evaluated in vitro as a single exposure on two consecutive days.29 A single dose of 5 J/cm2 resulted in increased proliferation and viability of injured fibroblasts. Higher doses (10 and 16 J/cm2) had reduced cell proliferation and viability, with damage to the cell membrane and DNA. The results of this in vitro study do not necessarily imply that similar findings would be present in vivo, but do suggest that there may not be a linear dose-response effect. A review of experimental studies related to laser treatment of wounds in 8 mouse and 39 rat model studies has been reported.30 Wound healing in rodent models differs from wound healing in people because of the loosely attached skin in rodents as compared with people, making wound contraction a significant factor in healing in rodents. The loosely attached skin of rodents may be similar to dogs and cats, and therefore mice and rats may be a useful model of wound healing to evaluate treatments for dogs and cats. Many studies continue to inadequately report laser parameters used in treatment protocols. In spite of these shortcomings, the results of these studies suggest that laser or monochromatic light is effective as a treatment for wounds when applied at appropriate doses. Coherence of light may not be an important factor when treating surface wounds. LEDs with monochromatic light appear to have effects on topical wounds, similar to those obtained with laser light. Wavelengths in both the visible red (630-685 nm) and infrared (700-1000 nm) typically stimulated wound healing in a variety of models. Some studies suggested that the best results were obtained when using 660-nm laser light in the early stages of wound healing, whereas 780 nm produced positive results throughout all stages of wound healing.31,32 In general, there was no clear association between outcomes and power levels used in the studies evaluated in this review.30 However, lack of standard experimental design, including total dose administered, made it difficult to draw conclusions. In those studies that reported energy density or when calculation was possible, there appeared to be a dose-response relationship.30 However, there may be a biphasic response, with positive effects seen with lower dosages, and inhibitory effects at higher dosages.30 Treatment with a total dose of 20 J/cm2 divided between 638 and 830 nm light produced better outcomes than using either wavelength alone, suggesting a possible effect of both superficial and deep treatments.33 However, two studies with infrared laser treatment using higher dosages had reduced stimulation34 or inhibitory effects.35 In one study of rats with circular wounds receiving a single 830-nm laser treatment with 1.3 J/cm2, more rapid epithelialization and wound contraction resulted compared with 3 J/cm2, although both treatments resulted in stimulation of healing compared with controls, and there was no difference between the treated groups by day 14.34 In a mouse wound model, 18 J/cm2 of 980-nm laser treatment had a beneficial effect on wound healing, whereas 36 J/cm2 was too aggressive and resulted in decreased healing.35 Laser therapy may also be beneficial for difficult wounds in metabolically compromised patients. Laser photostimulation accelerated wound healing in diabetic rats in one study.1 Diabetes was induced in male rats by streptozotocin injection and two 6-mm diameter circular wounds were created on either side of the spine. The left wound of each animal was treated with a 632.8 nm HeNe laser at a dose of 1 J/cm2 5 days per week until the wounds closed (3 weeks). There was a marginal increase of biomechanical properties of the laser-treated wounds, including an increase in maximum load (16%), stress (16%), strain (27%), energy absorption (47%), and toughness (84%) compared with control wounds. The amount of total collagen was significantly increased in laser-treated wounds. It was concluded that laser photostimulation promoted tissue repair by accelerating collagen production and promoting overall connective tissue stability in healing wounds of diabetic rats. HeNe lasers also improved wound healing by increasing collagen synthesis when corticosteroids or nonsteroidal antiinflammatory agents were administered to rats with surgical abdominal wounds.36 Laser treatment significantly increased collagen synthesis. One study reported 100 clinical cases with healing wounds treated with LLLT.37 There was a marked increase in collagen formation, increased vasodilation, and accelerated DNA synthesis. This researcher recommended 1 J/cm2 of laser treatment. A statistical metaanalysis was performed to determine the overall treatment effects of laser phototherapy on tissue repair.18 After performing a literature search, the effectiveness of laser treatment was calculated from each study using standard procedures. Thirty-four peer-reviewed papers met the inclusion criteria for tissue repair. There was a positive effect of laser phototherapy on tissue repair. Collagen formation, rate of healing, tensile stress and strength, time needed for wound closure, number and rate of degranulation of mast cells, and flap survival were improved with laser therapy. Laser treatment with a wavelength of 632.8 nm had the greatest effect, and 780 nm had the least. This metaanalysis concluded that laser therapy was an effective treatment for tissue repair. Another study reviewed the literature regarding the in vitro and in vivo effects of low-intensity laser therapy on the wound healing process, especially in diabetic patients.38 Although many of the in vivo studies lacked specific information on dosimetric data and appropriate controls, the data from appropriately designed studies indicated that LLLT should be considered as an adjunctive adjuvant therapy for refractory wound-healing disorders, including those experienced by diabetic patients. However, results from other controlled and blinded studies have been less clear regarding the efficacy of LLLT for the treatment of wounds.11 Studies in laboratory animals have suggested that LLLT may improve healing during the early stages of wound healing, but the effect may not result in improved total healing time.39,40 Another large review paper did not find unequivocal evidence that LLLT was beneficial for the treatment of wound healing.41 Bone and cartilage may also be affected by laser treatment. In one study of bone healing, rats received a defect in a femur.42 Rats were treated for either 12 sessions (4.8 J/cm2 per session, 28-day follow up) or three sessions (4.8 J/cm2 per session, 7-day follow up) with 40 mW 830 nm laser light. Treatments were applied three times per week, and two other groups served as untreated controls. Rats were sacrificed on either day 7 or 28 after surgery. Although there were significant differences between treated and control animals regarding the area of mineralized bone at 7 days, there were no differences at 28 days. The authors suggested that LLLT may have some effect on early bone repair. Another study of bone healing in rats compared ultrasound with laser.43 A GaAlAs laser (780 nm) was applied using 30-mW power and a dose of 112.5 J/cm2. The ultrasound group was treated with 1.5 MHz, at 30 mW/cm2. Both groups received 12 total treatments, with 5 treatments administered per week. Bones were harvested on day 20. Maximum load at failure was greatest in the laser-treated group. Ultrasound resulted in promotion of bone resorption at the osteotomy site, whereas laser caused bone formation in comparison with control osteotomies. The use of GaAlAs laser was compared with bone morphogenetic protein (BMP) and bovine organic bone graft in a rat femoral bone healing model.44 Rats were assigned to four groups: control; laser treatment; BMPs plus organic bovine bone graft; and BMPs plus organic bovine bone graft plus laser treatment. The laser-irradiated groups received treatments every 48 hours for seven treatments, beginning immediately after the surgical procedure. The laser therapy (830 nm, 40 mW) consisted of 16 J/cm2 per session divided equally over four points (4 J/cm2 each) around the defect. The subjects were sacrificed after 15, 21, and 30 days. There was increased deposition of collagen at 15 and 21 days, as well as increased bone trabeculae at the end of the experimental period in the irradiated animals versus the nonirradiated controls. The greatest healing was seen in the group treated with organic bovine bone graft, BMP, and laser treatment. LLLT has also been investigated as an adjunctive treatment for infections of the musculoskeletal system. In one study, the effect of various doses of 808 nm (100 mW continuous laser, at doses of 7.64 J/cm2, 15.29 J/cm2, and 22.93 J/cm2) laser therapy was evaluated in induced chronic osteomyelitis of rat tibias, created with methicillin-resistant Staphylococcus aureus.45 Rats had either surgical debridement, surgical debridement plus laser therapy, or no treatment. Infection levels decreased by 37%, 67%, 81%, and 93% in groups treated by debridement only, or debridement plus laser at 7.64 J/cm2, 15.29 J/cm2, or 22.93 J/cm2, respectively, compared with the nontreated control group. The authors concluded that laser therapy may be an adjunctive treatment for the management of osteomyelitis. Laser has also been used to create a laser shockwave to clear biofilm from medical devices in vitro.46 Bacteria surrounding medical devices, such as stents and orthopedic implants, may make a biofilm coating that prevents penetration of antibiotics. In this study, the biofilm was disrupted by a pulsed Nd:YAG laser that produced laser-generated shockwaves, making the bacteria susceptible to conventional treatment. A more complete review of the effects of laser therapy for bone repair has been published.47 Most of the research regarding bone healing has been performed in cell culture or in rodent models. However, the authors acknowledge that more study on the laser properties, wavelength, and energy dosage is needed, along with improved study design. The safety of laser therapy on epiphyseal cartilage has been evaluated in rats.48 Young rats had either no laser irradiation, 5 J/cm2, or 15 J/cm2 of 830 nm GaAlAs laser application to the proximal tibial epiphyseal cartilage every other day for a total of 10 sessions. Laser irradiation increased epiphyseal cartilage thickness and the number of chondrocytes, but these effects were not great enough to increase bone length. Another study evaluated osteochondral lesions of the knee treated intraoperatively with laser therapy in rabbits.49 Bilateral osteochondral lesions were created in the femoral medial condyles. All of the left lesions underwent immediate stimulation using a GaAlAs laser (780 nm), and the right knees were left untreated as a control group. After 24 weeks, the condyles were examined histomorphometrically. The condyle treated with laser had better cell morphologic findings and repair of osteocartilaginous tissue. The effect of laser therapy on cartilage was further investigated in another study that evaluated whether intraoperative laser biostimulation can enhance healing of cartilaginous lesions of the knee in rabbits.50 Bilateral chondral lesions were created in the medial femoral condyles. The lesion in the left knee of each animal was treated intraoperatively using a diode GaAlAs 780-nm laser (300 J/cm2, 1 W, 300 Hz, 10 minutes), and the right knee was left untreated. Cartilage was then examined 2, 6, or 12 weeks after surgery. The rabbits receiving laser therapy had progressive filling with fibrous tissue of the cartilaginous lesion, whereas no changes were apparent in the untreated group. Although laser therapy may have some benefit to treat cartilage injury, it may also have benefit to help maintain the health of cartilage during periods of disuse and immobilization. The influence of laser therapy (632.8 nm, HeNe, 13 J/cm2, three times a week) on the articular cartilage of rabbit stifles immobilized for 13 weeks was examined in one study.51 The number of chondrocytes and depth of articular cartilage of the treated rabbits were significantly higher than those of the sham-treated group. The cartilage surface of the sham-treated group was rough and fibrillated, whereas the surface of the experimental group was intermediate between a nonimmobilized control group and the sham-treated group. The authors of this study concluded that low-power HeNe laser irradiation reduced the adverse effects on the articular cartilage of rabbits immobilized for 13 weeks. Another study evaluated the use of 810 nm laser therapy on bone and cartilage during joint immobilization of rat knees.52 Three groups of rats received either 3.9 W/cm2, 5.8 W/cm2, or sham treatment. After 6 treatments over a 2-week period, tissues were harvested for testing. Results indicated that cartilage stiffness, assessed by indentation testing, was preserved in both laser groups.
Laser Therapy in Canine Rehabilitation
Laser Therapy
Properties of Lasers
Figure 21-1 Electromagnetic spectrum. Laser light typically falls within the infrared or near red portion of the electromagnetic spectrum. (Photo Courtesy Companion Laser, LiteCure LLC, Newark, Delaware.)
Figure 21-2 A, Sunlight, or white light, may be broken into several component colors with different wavelengths. B, Laser light is monochromatic and is produced by a single wavelength.
Figure 21-3 Laser light is coherent, with the photons traveling in the same phase and direction, whereas normal light is noncoherent.
Figure 21-4 Laser light is collimated, which means that there is minimal divergence in the laser beam over a distance. Normal light is not collimated, and the beam diverges over a distance.
Figure 21-5 The wavelength of the photons is important in laser therapy. Various substances preferentially absorb laser light at different wavelengths. (Photo Courtesy Companion Laser, LiteCure LLC, Newark, Delaware.)
Figure 21-6 Three different doses of laser light and penetration through skin. Greater doses of laser energy penetrate deeper into tissues. (From Topping A, Gault D, Grobbelaar A et al: Does Low Penetration of Human Skin by the Normal Mode Ruby Laser Account for Poor Permanent Depilatory Success Rates? Lasers Med Sci 16:224-229, 2001.)
Figure 21-7 Diagram of laser light delivered as continuous wave or pulsed laser light of various pulse durations. (Based on a diagram from Hashmi JT et al: Effect of pulsing in low-level light therapy. Lasers Surg Med 42:450-466, 2010.)
Classification of Lasers
Lasers Used in Physical Rehabilitation
AM Radio
10,000 cm
TV and FM
100 cm
Microwave
10 cm
Infrared
700 nm
Ultraviolet
10 nm
X-rays
1 nm
Biologic Effects of Laser Therapy
Research Regarding Laser Therapy
Wound Healing
Bone and Cartilage Effects
Stay updated, free articles. Join our Telegram channel
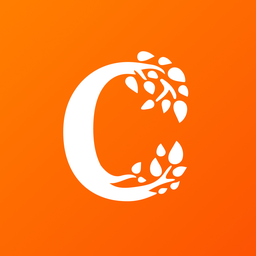
Full access? Get Clinical Tree
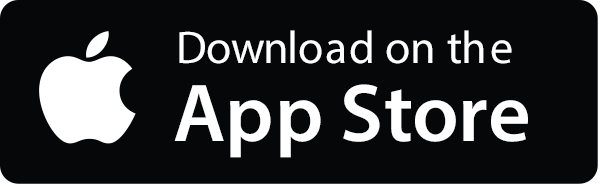
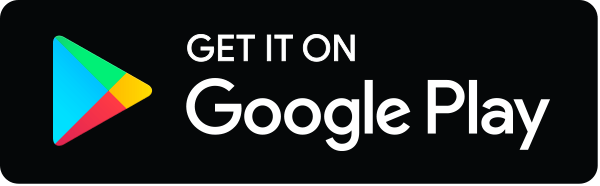