Chapter 9 Iron Metabolism and Its Disorders V. REGULATION OF IRON METABOLISM A. Intracellular Regulation of Iron Metabolism B. Systematic Regulation of Iron Metabolism A. Iron Metabolism in Erythroid Cells B. Iron Metabolism in Macrophages C. Iron Metabolism in Hepatocytes VII. TESTS FOR EVALUATING IRON METABOLISM C. Serum Total Iron-Binding Capacity F. Erythrocyte Zinc Protoporphyrin VIII. DISORDERS OF IRON METABOLISM B. Anemia of Inflammatory Disease F. Siderotic Inclusions in Erythroid Cells Iron is an essential nutrient required in a wide variety of metabolic processes. In solution, iron exists in two oxidation states, ferrous (Fe+2) and ferric (Fe+3), which can donate or accept electrons, respectively. Iron is typically in the Fe+2 state for transport across membranes and in the Fe+3 state when bound to transport and storage proteins. Iron may be in either oxidation state when present in heme and iron-sulfur (Fe-S) cluster-containing proteins. The ability of iron to vary in oxidation state and redox potential, depending on the ligands it forms, enables iron to serve multiple functions. Iron-containing proteins are critical for oxygen transport and storage, respiration, DNA synthesis, citric acid cycle function, and various enzymatic reactions. Unfortunately, the same physical characteristics that allow iron to function as a cofactor in controlled redox biochemistry also makes iron potentially toxic to cells, because of its ability to catalyze the formation of reactive oxygen species. The binding of iron to coordinating ligands, such as O, N, and S, in proteins and the tight regulation of iron uptake, transport, and storage tends to shield iron from reactions with molecular oxygen that generate free radicals and injure tissues (Ryan and Aust, 1992). Iron is absorbed from the diet in the small intestine and transferred to plasma, where it is bound to transferrin for transport to cells within the body. Once inside the body, iron cycles in a nearly closed system (Fig. 9-1) because little iron is lost in domestic animals unless hemorrhage occurs (Finch et al., 1978). About 75% of the iron present in plasma will be transported to the bone marrow for incorporation into hemoglobin in developing erythroid cells (Smith, 1997). The remaining plasma iron is taken up by nonerythroid tissues, primarily the liver (Koury and Ponka, 2004). Erythrocytes containing hemoglobin normally circulate for several months before being phagocytized by macrophages when senescent. After phagocytosis, erythrocytes are lysed, hemoglobin is degraded, and iron is released. Most iron from degraded hemoglobin is quickly released back into plasma, but a small amount may be stored as ferritin or hemosiderin within macrophages, which is released more slowly into plasma. The vast majority of iron entering plasma each day comes from macrophage release. Iron absorption from the small intestine compensates for the small amount of iron lost each day, largely from skin exfoliation and cell desquamation in urine, bile, sweat, and feces (Beutler, 2006b; Underwood, 1977). FIGURE 9-1 Iron cycle. Iron (Fe) is highly conserved in the body. Iron in plasma is bound to transferrin, a transport protein that is synthesized in the liver. Iron is transported to all tissues, but most iron is utilized to synthesize hemoglobin in developing erythroid cells. Aged blood erythrocytes are phagocytized by macrophages, and hemoglobin is degraded. Released iron is either returned to plasma or stored in macrophages as ferritin and hemosiderin. Nearly all of the iron in plasma under normal conditions comes from the release of iron by macrophages that have phagocytized and degraded erythrocytes (large arrow). Only about 3% of the iron in plasma results from gastrointestinal (GI) enterocyte absorption in normal individuals (small arrow). FIGURE 9-2 Overview of iron metabolism. Ferric iron (Fe+3) ions present in the diet are reduced to ferrous iron (Fe+2) ions and absorbed by duodenal enterocytes. Once inside enterocytes, Fe+2 may be oxidized and stored as ferritin or exported to plasma where it is oxidized and bound to transferrin (Tf) for transport to the tissues. Iron stored as ferritin is returned to the small intestine lumen when enterocytes are sloughed at the tip of the villus. Iron is incorporated into many proteins in all tissues, but most iron is utilized to synthesize hemoglobin in developing erythroid cells, where Fe+3 must be reduced to Fe+2 during heme synthesis. Aged blood erythrocytes are phagocytized by macrophages. Hemoglobin is degraded and iron is either returned to plasma or stored in macrophages as ferritin and hemosiderin. Modification of Kaneko, 1964, with permission. The relative distribution in the body is shown in Figure 9-2. Normally about 60% to 70% of total body iron is present in hemoglobin. About 20% to 30% is stored as ferritin and hemosiderin (primarily within macrophages and hepatocytes), 3% to 7% is present in myoglobin (with the higher values occurring in myoglobin-rich species such as dogs, horses, and cattle), 1% is present in enzymes, and less than 0.1% is bound to transferrin in plasma (Ponka et al., 1998). Hemoglobin accounts for more than 90% of the protein within erythrocytes (Quigley et al., 2004). It contains 0.34% iron by weight; consequently, each milliliter of packed erythrocytes contains 1.1 mg of iron. Hemoglobin plays vital roles in oxygen transport, carbon dioxide transport, and buffering of hydrogen ions (see Chapter 7 for details). It is a tetrameric protein consisting of four polypeptide globin chains, each of which contains a heme prosthetic group. Heme is a planar molecule composed of the tetrapyrrole protoporphyrin IX, containing a central iron ion. A hemoglobin tetramer is capable of binding four molecules of oxygen when fully saturated. To bind oxygen, iron ions within heme molecules must be in the Fe+2 state. Methemoglobin is formed when iron is oxidized to the Fe+3 state. Fortunately, the oxidation of Fe+2 ions to Fe+3 ions is minimized by the location of heme groups within hydrophobic pockets of globin chains, and methemoglobin that forms can be reduced enzymatically within erythrocytes. Ferritin is a ubiquitous iron storage protein that is detected in almost all animal and plant tissues, as well as in bacteria and fungi. The binding of iron in ferritin minimizes its potential to catalyze the formation of damaging free radicals (Arosio and Levi, 2002). Each mammalian ferritin molecule is composed of a protein shell of 24 apoferritin H or L monomeric subunits surrounding a central cavity that can accommodate as many as 4000 iron atoms in a ferric hydroxide core. The inner surface of the protein shell has catalytic sites (associated with H subunits) that promote the oxidation of Fe+2 ions to Fe+3 ions (Arosio and Levi, 2002). The L subunit lacks ferroxidase activity, but it is more efficient than the H subunit in the enucleation and mineralization of iron in the ferritin core (Levi et al., 1994). Subunit composition varies between cell types, with the H subunit predominating in erythrocytes and muscle, and the L subunit predominating in liver, spleen, and plasma of humans (Beutler, 2006b). Dog and horse ferritin H and L subunit cDNA clones have been prepared and sequenced from various tissues. The amino acid residues involved in the ferroxidase center and in iron nucleation were conserved in H and L subunits of canine and equine ferritins, respectively (Orino et al., 2005). Although iron is generally stored in cells as ferritin, iron overloaded cells (macrophages in normal animals) contain another storage form of iron called hemosiderin, which represents partially degraded ferritin that forms after uptake by lysosomes (Anderson and Frazer, 2005; Ponka et al., 1998). Surprisingly little is known about how ferritin releases its iron in cells. It is possible that intracellular iron release requires catabolism in lysosomes (Ponka et al., 1998). Free cytoplasmic ferritin molecules are water soluble and visible by electron microscopy, but they are not visible by light microscopy unless present in large aggregates. Hemosiderin is insoluble and visible by light microscopy. Hemosiderin appears gray to black in aspirate smears stained with routine bloodstains, golden-brown in H & E-stained tissue sections, and blue when stained with Prussian blue stain. There is a fairly good correlation between Prussian blue staining of tissues and the measurement of storage iron chemically, but staining is less sensitive in detecting storage iron (Blum and Zuber, 1975; Franken et al., 1981). Storage iron can be present as ferritin that is not identified by Prussian blue staining (Blum and Zuber, 1975). Major sites of iron storage include the liver, spleen, and bone marrow. The amount stored in a given tissue may vary by species. The liver appears to be the major site of iron storage in humans, but the spleen is reported to be more important in horses and cattle (Blum and Zuber, 1975; Franken et al., 1981; Kolb, 1963). The relative contributions of ferritin and hemosiderin to the storage iron pool vary with species, organ, and amount of iron stored. In normal adults, ferritin concentration generally equals or exceeds hemosiderin concentration in the liver, but hemosiderin concentration typically exceeds ferritin concentration in the spleen. When iron stores are high, hemosiderin tends to accumulate more than ferritin, and when iron stores are low, ferritin tends to be higher than hemosiderin (Kolb, 1963; Underwood, 1977). Total body iron stores vary at birth between species, with considerable iron stores in humans, dogs, cattle, goats, horses, and rabbits; intermediate iron stores in cats and rodents; and minimal iron stores in pigs (Keen et al., 1981; Kolb, 1963; Underwood, 1977). Iron stores decrease in nursing animals when demands for growth exceed iron absorption from milk (Blum and Zuber, 1975; Harvey et al., 1987a; Keen et al., 1981; Kolb, 1963). Myoglobin is an oxygen-binding protein located primarily in muscles. It contains one heme group per molecule and has a structure similar to that of hemoglobin monomers. Myoglobin serves as a local oxygen reservoir that can temporarily provide oxygen when blood oxygen delivery is insufficient during periods of intense muscular activity. Iron within the heme group must be in the Fe+2 state to bind oxygen. If iron is oxidized to the Fe+3 state, metmyoglobin is formed. The total amount of myoglobin in an animal depends on body weight, degree of muscle development, and the myoglobin concentration in muscle, which varies between muscle types (red muscle is rich in myoglobin and white muscle is myoglobin poor). The myoglobin concentration in muscle varies with species. For example, the myoglobin concentration in muscles of racehorses is about 6 times that of human muscles (Kolb, 1963). Because much of the iron in muscle is in myoglobin, the percentage of total body iron present in muscle is higher in horses than in humans. In addition to the need for iron in hemoglobin and myoglobin, iron is vital in various metabolic processes. Nearly half of the enzymes in the citric acid cycle either contain iron or need it as a cofactor. Heme-containing proteins include catalase, peroxidase, and cytochromes. Cytochromes a, b, and c are required for oxidative phosphorylation in mitochondria. Other cytochromes, such as cytochrome P450 located in the endoplasmic reticulum, function in oxidative degradation of endogenous compounds and drugs. Nonheme iron, in Fe-S compounds and metalloflavoproteins, is required for enzymes including succinate dehydrogenase, cytochrome c reductase, nicotinamide adenine dinucleotide dehydrogenase, xanthine oxidase, and aconitase (Smith, 1997). Nearly all of the iron present in plasma in healthy animals is bound to apotransferrin, a 72 to 83 kDa glycoprotein (containing 2 to 4 sialic acid residues/molecule) to form transferrin (Welch, 1990). Apotransferrin is a bilobar protein with two binding sites for Fe+3 that is synthesized by the liver. It is believed to have evolved from duplication of a primordial gene coding for a protein with one iron-binding site. The concomitant binding of a bicarbonate anion is required for each Fe+3 molecule bound to apotransferrin (Ponka et al., 1998). Normally 25% to 50% of the plasma apotransferrin binding sites are saturated with iron. When one or two Fe+3 ions are bound, the protein is referred to as monoferric or diferric transferrin, respectively. There is a random distribution of iron on binding sites (Fig. 9-3), with apotransferrin predominant at low plasma iron concentrations and diferric transferrin predominant at high plasma iron concentrations (Huebers et al., 1984). Monoferric transferrin is predominant at 50% saturation of transferrin with iron, with lesser amounts of monoferric and diferric iron present. Transferrin is primarily responsible for iron transport throughout the body. FIGURE 9-3 Apotransferrin is a bilobar protein with two binding sites for Fe+3 ions. When one or two Fe+3 ions are bound, the protein is referred to as monoferric or diferric transferrin, respectively. There is a random distribution of iron on binding sites, with apotransferrin predominant at low plasma iron concentrations and diferric transferrin predominant at high plasma iron concentrations. Monoferric transferrin is predominant at 50% saturation of transferrin with iron, with lesser amounts of apotransferrin and diferric transferrin present. FIGURE 9-4 Mechanisms of iron absorption. Ferrous iron (Fe+2) ions are transported into enterocytes in the duodenum by the divalent metaltransporter-1 (DMT1) after reduction of ferric iron (Fe+3) ions using a duodenal cytochrome b (DctyB). Heme is transported into enterocytes using heme carrier protein-1 (HCP1). Once inside, inorganic iron is released from heme by the action of the heme oxygenase (HO) reaction. Fe+2 ions are exported from enterocytes using ferroportin, oxidized to Fe+3 using hephaestin, and bound by apotransferrin (aTf) to form monoferric transferrin (mTf) and diferric transferrin (not shown). Hepcidin in plasma inhibits iron export to plasma by interacting directly with ferroportin, leading to ferroportin’s internalization and lysosomal degradation. Fe+2 not transported to plasma is stored as ferritin following oxidation to Fe+3. Iron stored as ferritin is returned to the small intestine lumen when enterocytes are sloughed at the tip of the villus. Ferritin is another iron-binding protein that can be measured in plasma. Although ferritin is released in small amounts from the liver and macrophages and can be taken up by cells (especially hepatocytes), it is of little or no importance in iron transport under normal conditions. Ferritin is normally present in very low concentrations in plasma and has low iron content; consequently, it contributes little to the plasma iron pool (Ponka et al., 1998). Nontransferrin bound iron (NTBI) has been measured in people with severe iron overload, when transferrin is fully saturated, and in mice with hereditary hypotransferrinemia. The nature of NTBI is unclear, but it may be complexed with low-molecular-weight molecules such as citrate, sugars, and amino acids or nonspecifically bound to albumin and other plasma proteins. NTBI has the potential to promote the formation of oxygen-free radicals and cause tissue injury (Ponka et al., 1998). Iron is not actively excreted from the body and minimal iron loss normally occurs, except in menstruating primates and egg-laying birds (Finch et al., 1978; Kolb, 1963); consequently, the amount within the body must be controlled at the point of absorption. Iron absorption from the diet depends on age, species of the animal, iron stores, changes in rate of erythropoiesis, hypoxia, inflammation, and pregnancy, as well as the amount and chemical form of iron ingested (Frazer and Anderson, 2005; Mackenzie and Garrick, 2005; Stewart et al., 1953). A low percentage (0.2% to 4.5%) of dietary iron is generally absorbed in normal adult animals (Finch et al., 1978; Nathanson et al., 1985). Iron absorption occurs through mature villus enterocytes of the duodenum and proximal jejunum. Absorption includes iron uptake at the apical membrane of the enterocyte, translocation within the cytoplasm, and transfer to plasma at the basolateral membrane of the enterocyte. Iron can be taken in by enterocytes as free ions or as heme by different pathways (Fig. 9-4). The relative importance of these pathways varies depending on animal species and diet (Steele et al., 2005). Compounds in the diet such as phytates, tannins, and phosphates bind iron in insoluble complexes that cannot be absorbed. Most nonheme iron in the diet is in the Fe+3 state. Dietary Fe+3 is solubilized from food by hydrochloric acid in the stomach and binds to mucins and various small molecules, which keep the iron soluble and available for absorption in the more alkaline environment of the small intestine (Mackenzie and Garrick, 2005). Unbound Fe+3 is prone to hydrolysis, forming essentially insoluble ferric hydroxide and oxohyroxide polymers (Ponka et al., 1998). The most important pathway for nonheme iron uptake utilizes the divalent metal transporter-1 (DMT1). A proton-coupled process facilitates this uptake. Although DMT1 has the highest affinity for iron, it can also transport manganese and potentially other divalent metal ions. Fe+3 ions must be reduced to Fe+2 ions before they can be transported into the enterocyte via the DMT1. Ascorbic acid in the diet or from gastric or biliary secretions promotes the reduction of Fe+3 ions. Although some Fe+3 ion reduction may occur by direct interaction with ascorbic acid, most reduction appears to rely on the presence of one or more brush border ferrireductase enzymes. A duodenal cytochrome b (DcytB) is believed to be important in this regard, but other intestinal ferrireductases may also exist (Mackenzie and Garrick, 2005). Although the dominant role of DMT1 is acknowledged, debate continues as to whether important alternative pathways of nonheme iron uptake exist. The role of the protein mobilferrin needs further evaluation. It might be part of a separate uptake pathway for Fe+3 ions, or it might interact with DMT1 in Fe+2 ion uptake (Conrad et al., 2000; Mackenzie and Garrick, 2005). Although humans absorb Fe+2 salts more readily from the intestine than the Fe+3 salts, dogs are reported to absorb both valence forms equally well (Moore et al., 1944). The reason for this difference is not known, but it could be related to a greater ability to reduce Fe+3 in the dog intestine, the presence of an important pathway for Fe+3 absorption in the dog intestine, or a greater ability to prevent the formation of insoluble Fe+3 complexes in the gastrointestinal tract of dogs. Heme is released from dietary myoglobin and hemoglobin by the action of digestive enzymes. Dietary heme iron is generally more bioavailable than is nonheme iron and is an important nutritional source of iron in carnivores and omnivores. Heme enters duodenal enterocytes as an intact metalloporphyrin using a membrane protein named heme carrier protein 1 (HCP1) that has homology to bacterial metal-tetracycline transporters (Shayeghi et al., 2005). The expression of HCP1 is regulated pre- and posttranslationally in hypoxic and iron-deficient mice, respectively (Latunde-Dada et al., 2006b). Once inside the enterocyte, inorganic iron is apparently released from heme by the action of the heme oxygenase reaction, and this heme-splitting reaction appears to be the rate-limiting step in the absorption of iron contained within hemoglobin and myoglobin (Wheby and Spyker, 1981). The intracellular transport of iron within the enterocyte is poorly understood. Iron ions in this labile iron pool (LIP) are presumably bound to chaperone molecules to keep them soluble. The nature of these chaperone molecules is yet to be defined. Iron ions can catalyze oxidative reactions that would injure the cell. The enterocyte protects itself against the toxic effects of excess iron by increasing apoferritin synthesis and incorporating the excess iron into ferritin. Consequently, iron uptake into enterocytes in excess of that needed for metabolic purposes or transferred to plasma is stored as ferritin. Iron stored as ferritin is returned to the small intestine when enterocytes are sloughed at the tip of the villus after 1 to 2 days (Steele et al., 2005). The transfer of iron atoms from enterocytes to transferrin in plasma is mediated by ferroportin (also known as Ireg1), an iron transport protein located on the basolateral surface of mature enterocytes. In addition to ferroportin, the efflux of iron from enterocytes requires a copper-containing protein called hephaestin that is also located on the basolateral membranes of mature enterocytes. Hephaestin is a membrane-bound ferroxidase that has significant homology to the plasma protein ceruloplasmin. Hephaestin’s function may relate to its ability to oxidize Fe+2 ions to Fe+3 ions for binding to transferrin in plasma (Steele et al., 2005). Sex-linked anemia (sla) mice have a block in mucosal iron transfer to plasma, with resultant microcytic hypochromic anemia because these animals have an inherited hephaestin defect (Wessling-Resnick, 2006). Components of brush border iron uptake, including DMT1 and DcytB, are strongly influenced by the iron concentration within enterocytes, with increased components expressed when intracellular iron content is low and decreased components expressed when iron content is high (Frazer and Anderson, 2005). These locally responsive changes in brush border transport components help buffer the body against the absorption of excessive iron, but it is the control of the basolateral transport of iron from enterocytes to plasma that represents the primary site at which iron absorption is controlled (Steele et al., 2005). Hepcidin, a peptide produced by hepatocytes and secreted into plasma, inhibits iron transfer from enterocytes to plasma by interacting directly with ferroportin, leading to the internalization and lysosomal degradation of this iron export protein. Hepcidin production is increased in disorders such as iron overload and inflammation that result in decreased intestinal iron absorption. Hepcidin production is decreased in iron deficiency and disorders with increased erythropoiesis that result in increased iron absorption by enterocytes. Hephaestin expression is minimally affected by iron status (Vokurka et al., 2006). At physiological pH and oxygen tension, Fe+2 in solution is readily oxidized to Fe+3, which is prone to hydrolysis and precipitation. In addition, unless appropriately chelated, iron in plasma can promote harmful oxygen radical formation and peroxidative tissue damage because of its catalytic action in one-electron redox reactions (Ponka et al., 1998). Fortunately, most iron in plasma is bound to the protein apotransferrin to form transferrin. The binding of iron to apotransferrin keeps iron molecules soluble and prevents iron catalyzed oxidative reactions. In addition, the vast majority of iron is transported to cells within the body following binding to apotransferrin in plasma (Ponka et al., 1998). At normal iron saturation levels, apotransferrin is most abundant in plasma, followed by monoferric transferrin and diferric transferrin (Ponka et al., 1998). Although diferric transferrin is normally much lower in concentration (about 10% of total transferrin) than monoferric transferrin, it accounts for most of the iron delivery to cells because diferric transferrin binds with 8- to 10-fold higher affinity to transferrin receptor-1 (TfR1) receptors on cells than does monoferric transferrin (Anderson and Frazer, 2005; Huebers et al., 1985). Apotransferrin has very low affinity for TfR1 receptors on cells (Ponka et al., 1998). Iron turns over in 3 h or less in plasma (Smith, 1997). Nearly all of the iron in plasma under normal conditions comes from the release of iron by macrophages that have phagocytized and degraded erythrocytes (Fig. 9-1). In contrast, only about 3% of the iron in plasma results from enterocyte absorption in normal individuals (Ponka et al., 1998). The synthesis of a number of proteins important in iron metabolism, including apotransferrin, TfR1, apoferritin, DMT1, DcytB, ferroportin, iron responsive protein-1 (IRP1), and erythroid-specific 5-aminolevulinic acid synthase (eALAS or ALAS2), is regulated posttranscriptionally depending on intracellular iron content (Beutler, 2006b; Koury and Ponka, 2004; Latunde-Dada et al., 2006a). The mRNA for each protein contains one or more iron responsive elements (IREs), each consisting of a stem-loop structure. The IREs located at the 5′ end of mRNAs regulate translation, and IREs located at the 3′ end regulate mRNA stability. IRP1 contains an Fe-S (4Fe-4S) cluster and exhibits aconitase activity when cells are iron replete, but it lacks the Fe-S cluster and aconitase activity when cytoplasmic iron is scarce. IRP1 binds tightly to IREs when cytoplasmic iron content is low. IRP2 is closely related to IRP1, but it lacks aconitase activity. The regulation of IRP2 is mediated by proteosomal degradation when cellular iron is adequate, through binding to iron and possibly heme (Wingert et al., 2005). The binding of IRPs to IREs at the 5′ end of mRNAs (including apoferritin and eALAS) inhibits translation and protein synthesis from these mRNAs, but the binding of IRPs to IREs at the 3′ end of mRNAs (including TfR1 and intestinal DMT1) promotes mRNA stability, thereby enhancing protein synthesis from these mRNAs. When cytoplasmic iron content is high, IRPs are displaced from IREs, resulting in opposite effects on protein synthesis (Starzynski et al., 2004). Because of these controlling factors, TfR1 synthesis is higher and apoferritin synthesis is lower when cytoplasmic iron content is low, and TfR1 synthesis is lower and apoferritin synthesis is higher when cytoplasmic iron content is high (Napier et al., 2005). Mitochondria are clearly central in the intracellular metabolism of iron; however, much remains to be discovered about their iron metabolism. The first enzyme reaction in heme synthesis (ALAS) and the final three enzyme reactions (including the insertion of Fe+2 into protoporphyrin IX by ferrochelatase to form heme) occur within mitochondria (see Chapter 8 for details), and proteins involved in Fe-S cluster synthesis are located within mitochondria. Fe-S cluster synthesis has been studied in microbes, but this pathway has not been well characterized in mammals. An inherited defect in a small protein called frataxin causes Friedreich ataxia in humans (Napier et al., 2005). This disorder results in spino/cerebellar ataxia, cardiomyopathy, and diabetes associated with mitochondrial iron overload in nerve and cardiac tissue, but not in erythroid cells. Frataxin participates in the synthesis of Fe-S clusters, possibly as a mitochondrial iron chaperone that shields this metal from reactive oxygen species and renders it bioavailable as Fe+2 (Napier et al., 2005). Heme and Fe-S clusters synthesized within mitochondria are used locally and exported from mitochondria for insertion into cytoplasmic and nuclear enzymes. With the exception of a limited number of enzymes, such a ribonucleotide reductase, that have mono- or di-iron-binding sites, iron in heme and Fe-S clusters accounts for most of the enzyme iron. Consequently, almost all of the iron within cells must transit through mitochondria to be functionally active (Arosio and Levi, 2002). Mitoferrin, a member of the vertebrate mitochondrial solute carrier family (SLC25), appears to be essential for the uptake of iron into mitochondria (Shaw et al., 2006). The transporter needed to export heme from mitochondria to the cytoplasm is unknown; however, an inner mitochondrial membrane ATP-binding cassette protein B7 (ABCB7) appears to be required as an exporter for Fe-S clusters (Napier et al., 2005). Studies in a zebra fish shiraz model indicate that heme synthesis in erythroid cells is dependent on the production of Fe-S clusters (Wingert et al., 2005). Deficient Fe-S cluster formation (resulting from glutaredoxin 5 deficiency) and the resultant deficiency of Fe-S clusters in the cytoplasm of developing erythroid cells result in IRP1 binding to IREs of eALAS and the inhibition eALAS translation. A deficiency in eALAS activity in zebra fish erythroid cells results in deficient heme synthesis and the formation of hypochromic erythrocytes (Wingert et al., 2005). A human with mitochondrial glutaredoxin 5 deficiency has recently been described with sideroblast-like microcytic anemia and iron overload (Camaschella et al., 2007). Mitochondria can store excess iron using mitochondrial ferritin (m-ferritin). Unlike cytoplasmic apoferritin, mitochondrial apoferritin lacks an IRE and may be transcriptionally regulated by iron. M-ferritin forms homopolymeric shells that have more homology with cytoplasmic H-ferritin chains than L-ferritin chains (Napier et al., 2005). M-ferritin concentration is very low in most cells, including erythroid cells, but it is markedly increased in disorders with mitochondrial iron overload, presumably limiting the oxidative damage generated by the excess iron. M-ferritin appears to be degraded to hemosiderin-like material within mitochondria, but the mechanism is unknown (Napier et al., 2005). Although all cells require iron, erythroid precursors have iron requirements that far exceed the iron requirements of any other cell type because of the need for hemoglobin synthesis. Erythrocytes contain approximately 45,0000-fold more heme iron (largely in hemoglobin) than nonheme iron, and the delivery of iron to erythrocytes is stringently dependent on transferrin as the source of iron (Koury and Ponka, 2004). Diferric transferrin molecules bind to TfR1 on the surface of cells, and these transferrin-TfR1 complexes localize to clathrin-coated pits, which invaginate to initiate endocytosis (Beutler, 2006b). Clathrin is a structural protein that enables endocytosis through the formation of concave lattices with the plasma membrane. After the transferrin-TfR1 complexes are internalized as endosomes, a proton pump decreases the pH in the endosome, resulting in conformational changes in the proteins and subsequent release of Fe+3 ions from transferrin (Fig. 9-5). Iron is exported from the endosome using DMT1, following reduction with Steap3, an NADPH-dependent ferrireductase (Ohgami et al., 2005). The resultant apotransferrin-TfR1 complex is recycled to the cell membrane, where apotransferrin is released from the cell, and the TfR1 is again available for binding additional iron-containing transferrin molecules. Erythroid precursor cells in the bone marrow and reticulocytes that synthesize hemoglobin have TfR1 on their surfaces for iron uptake, but reticulocytes lose their TfR1 as they develop into mature erythrocytes (Ponka et al., 1998). FIGURE 9-5 Iron metabolism in erythroid cells. Diferric transferrin (dTf) binds to transferrin receptor-1 (TfR1) on the surface of developing erythroid cells. The dTf-TfR1 complexes are internalized within an endosome and a proton pump decreases the pH in the endosome, resulting in release of iron ions (dark spheres). Iron is exported from the endosome using the divalent metal transporter-1 (DMT1). The resultant apotransferrin (aTf)-TfR1 complex is recycled to the cell membrane, where aTf is released from the cell, and the TfR1 is available for binding additional dTf. A direct interorganelle transfer of iron occurs between endosomes and mitochondria for insertion into protoporphyrin IX (PP) to form heme and for synthesis of iron-sulfur clusters (not shown). Some iron presumably exists in a cytoplasmic labile iron pool (LIP), with excess iron stored as ferritin. A direct interorganelle transfer of iron occurs between endosomes and mitochondria where iron is used for heme and iron-sulfur cluster synthesis (Sheftel et al., 2007). Some iron is presumably released from endosomes into a cytoplasmic labile iron pool (LIP), with excess cytoplasmic iron stored as ferritin. Various chaperone molecules have been proposed, but the nature of the iron in the LIP remains enigmatic (Beutler, 2006b). Iron stored as ferritin in the cytoplasm is not available for hemoglobin synthesis in erythroid cells (Ponka et al., 1998). TfR1, apoferritin, and eALAS synthesis are regulated by the amount of intracellular iron present. An increase in the LIP stimulates apoferritin synthesis and inhibits TfR1 expression to minimize the potential of iron toxicity to the cell. A decrease in the LIP results in decreased apoferritin synthesis and increased TfR1 expression on cell surfaces to maximize iron uptake and use for heme synthesis (Beutler, 2006b). Erythrocytes coordinate protoporphyrin IX formation with the availability of iron by increasing the synthesis of eALAS (rate limiting enzyme in porphyrin synthesis) when the LIP is high, and decreasing eALAS synthesis when the LIP is low. Even though three different pathways are required for hemoglobin synthesis in erythrocyte precursors and reticulocytes, virtually no intermediates (iron, globin chains, or heme) accumulate in the cytoplasm of these cells. Several positive and negative feedback mechanisms account for the balanced production of these hemoglobin components. As already discussed, an increase in the LIP limits the uptake of additional iron by decreasing TfR1 synthesis. The availability of iron also limits, and thereby controls, heme synthesis. Free “uncommitted” heme inhibits iron uptake by erythroid cells and consequently heme synthesis. In addition, free heme is essential for the synthesis of globin chains at both the transcriptional and translational levels (Koury and Ponka, 2004). Consequently, globin synthesis does not occur in the absence of heme. A heme exporter termed FLVCR is up-regulated on colony-forming units-erythroid (CFU-E) progenitor cells. It may provide a safety mechanism to prevent the accumulation of toxic amounts of cytoplasmic heme before globin synthesis is initiated. FLVCR is the cell surface receptor for feline leukemia virus, subgroup C (FeLV-C). Cats with FeLV-C infections develop erythroid aplasia because of a block at the CFU-E stage of erythroid development. It appears that the binding of FeLV-C to receptors on CFU-E progenitor cells inhibits heme export and results in apoptosis of these cells (Quigley et al., 2004). In addition to the entry of iron into mitochondria for incorporation into protoporphyrin IX to form heme, iron is critical for Fe-S cluster biogenesis within mitochondria. Fe-S clusters are important prosthetic groups for numerous proteins involved in electron transfer, metabolic, and regulatory processes. Although Fe-S clusters are formed within mitochondria, Fe-S proteins are located in the nucleus and cytoplasm of cells, as well as in mitochondria (Lill et al., 2006). Recent studies in zebra fish provide evidence for a regulatory link between Fe-S cluster formation and heme synthesis. In the absence of Fe-S clusters, IRP1 binds to eALAS mRNA, which inhibits eALAS synthesis and heme production (Wingert et al., 2005). In contrast to erythroid cells, virtually no iron enters macrophages via plasma transferrin. Rather, nearly all iron enters macrophages by the phagocytosis of aged or prematurely damaged erythrocytes (Fig. 9-6) (Ponka and Richardson, 1997). Following phagocytosis, erythrocytes are lysed, and hemoglobin is degraded to heme and globin. The microsomal heme oxygenase reaction within macrophages degrades heme and releases iron. Most of the iron from degraded heme is quickly exported (half-life 34 min in dogs) from the macrophage and bound to plasma transferrin for transport to other cells (especially erythrocyte precursors in the bone marrow). The export of iron from macrophages is mediated by ferroportin and controlled by hepcidin as has been discussed for enterocytes (Verga Falzacappa and Muckenthaler, 2005). In contrast to enterocytes that utilize membrane-bound hephaestin, macrophages utilize the copper-containing plasma protein ceruloplasmin to oxidize Fe+2 ions to Fe+3 ions for binding to transferrin in plasma (Nemeth et al., 2004b). Iron not rapidly released to plasma is stored within macrophages as ferritin and hemosiderin. Iron that is stored within macrophages is released to plasma more slowly, with a half-life of 7 days in dogs (Fillet et al., 1974). The mononuclear phagocyte system accounts for much of the total body iron stores. Iron in the storage pool turns over slowly unless there is an increased need for iron for hemoglobin synthesis (Beutler, 2006b). In addition to iron export by ferroportin, a small amount of iron is apparently released as ferritin. It is not clear whether this is secreted or simply represents a “leak” from damaged cells (Ponka et al., 1998). FIGURE 9-6 Iron metabolism in macrophages. Nearly all iron enters macrophages by the phagocytosis of aged or prematurely damaged erythrocytes. Following phagocytosis, erythrocytes are lysed, and hemoglobin is degraded to heme and globin. The microsomal heme oxygenase reaction within macrophages degrades heme and releases iron to the labile iron pool (LIP). Most of the released iron is exported from the macrophage by ferroportin as ferrous iron, oxidized to ferric iron by ceruloplasmin (Cp) in plasma, and bound to apotransferrin (aTf) to form monoferric transferrin (mTf) or diferric transferrin (not shown). Hepcidin in plasma inhibits iron export by interacting directly with ferroportin, leading to ferroportin’s internalization and lysosomal degradation. Iron not rapidly released to plasma is stored within macrophages as ferritin, which may be degraded to hemosiderin within lysosomes. Macrophages can take up iron present in hemoglobin-haptoglobin complexes and heme-hemopexin complexes in plasma. Haptoglobin-hemoglobin complexes form following intravascular hemolysis when released hemoglobin binds with high affinity to the plasma glycoprotein haptoglobin. Heme-hemopexin complexes can also form secondary to intravascular hemolysis when hemoglobin heme is released, but additional heme-containing proteins (such as myoglobin) release heme that binds to the plasma protein hemopexin. The hemoglobin-haptoglobin complex undergoes endocytosis after binding to the hemoglobin scavenger receptor CD163. Expression of this receptor is induced by interleukin-6 (IL-6), IL-10, and glucocorticoids (Graversen et al., 2002). The heme-hemopexin complexes undergo endocytosis after binding to CD91, also called the low-density lipoprotein receptor-related protein (LRP) or α2-macroglobulin receptor. Once inside the cell, the complexes are transported to lysosomes for degradation, and receptors are recycled to the cell surface (Hvidberg et al., 2005). The liver is essential for normal iron homeostasis in the body. It regulates iron movement into and around the body through hepcidin synthesis, accounts for about 40% of the body iron stores, and is the site of synthesis of apotransferrin, ceruloplasmin, haptoglobin, and hemopexin plasma proteins (Anderson and Frazer, 2005). Hepatocytes synthesize hepcidin in an endocrine manner to regulate iron metabolism in the body. Hepcidin is a small cationic peptide (25 amino acids in humans) that forms from a larger prohepcidin peptide (84 amino acids) (Park et al., 2001). Hepcidin has been cloned, expressed, and sequenced in several species, including dogs (Fry et al., 2004; Verga Falzacappa and Muckenthaler, 2005). Because of its small size, hepcidin is rapidly cleared by the kidney and is measurable in urine. Using immunohistochemistry techniques, prohepcidin is localized to organelles of the secretory pathway, particularly the Golgi apparatus, of hepatocytes suggesting that molecules may accumulate before receiving a signal for secretion (Wallace et al., 2005). Prohepcidin circulates in plasma, but its plasma concentration does not correlate well with urinary hepcidin concentrations or other iron parameters (Kemna et al., 2005). The site, nature, and potential regulation of the conversion of prohepcidin to hepcidin remain to be identified. A number of systemic stimuli, including body iron stores, anemia, hypoxia, degree of erythropoiesis, and inflammation have been reported to modulate hepcidin levels; however, the pathways involved in hepcidin production remain to be elucidated (Anderson and Frazer, 2005; Steele et al., 2005). Anemia, hypoxia, and erythropoietin themselves do not seem to be of primary importance (Pak et al., 2006; Vokurka et al., 2006). Hepcidin production is modulated by body iron requirements, which are largely influenced by the magnitude of erythropoiesis present (Wilkins et al., 2006). A signal arising from erythropoietic activity in bone marrow is proposed to regulate hepcidin production, but its nature is unknown at the time of this manuscript preparation (Pak et al., 2006). The content of diferric transferrin in plasma may be a key indicator of body iron requirement (Wilkins et al., 2006). Many questions remain concerning how hepcidin expression is modulated in response to body iron requirements; however, three molecules (hemochromatosis [HFE] protein, TfR2, and hemojuvelin) have been identified that are involved in the regulation of this pathway(s). Defects in each of these molecules in humans or mice have resulted in inappropriately low hepcidin levels and increased iron absorption leading to hemochromatosis. It is hypothesized that hepcidin is regulated by HFE and TfR2 on hepatocyte surfaces in response to plasma transferrin saturation. TfR2 has a 25-fold lower affinity for diferric transferrin than does TfR1 and would presumably become active when diferric transferrin concentrations are high (Anderson and Frazer, 2005). The pathway involving plasma diferric transferrin concentration may not be the only way hepcidin production is regulated. Hemojuvelin may modulate this pathway or affect hepcidin synthesis independently (Steele et al., 2005). Studies indicate that hepcidin synthesis is stimulated by certain bone morphogenetic proteins (BMPs) that bind to hemojuvelin, regulating hepcidin expression through a BMP signal transduction pathway (Truksa et al., 2006). In addition, hepcidin expression increases in response to inflammation, and this increase precedes any alteration in transferrin saturation (Steele et al., 2005). Most of the iron stored within the liver is found in hepatocytes stored as ferritin. Hepatocytes secrete a glycosylated form of ferritin into plasma (Ghosh et al., 2004). Ferritin in human plasma is primarily composed of L subunits, but H subunits predominate in ferritin in dog plasma (Watanabe et al., 2000). Plasma ferritin concentrations generally correlate with body iron stores; however, ferritin is an acute phase protein that increases in plasma during inflammation (Torti and Torti, 2002). Hepatocyte membranes contain ferritin receptors. However, serum ferritin is present in low concentrations and contains little iron, so it is unlikely that hepatocytes take up significant amounts of iron from plasma by this mechanism under normal conditions (Anderson and Frazer, 2005; Ponka et al., 1998). Like other cells, hepatocytes utilize TfR1 to transport iron into the cells. NTBI is thought to be chelated by small organic acids, such as citrate, but some may be bound loosely to proteins such as albumin (Anderson and Frazer, 2005). The liver rapidly clears NTBI. In experimental studies using mice, the half-time of clearance of NTBI in plasma was 30 sec, compared to a half-time clearance for transferrin-bound iron of 50 min (Craven et al., 1987). Like macrophages and enterocytes, hepatocytes utilize ferroportin to export iron to plasma. Ferroportin expression is lower in hepatocytes than in enterocytes and macrophages, which may help explain why these cells preferentially accumulate iron in most iron-overload conditions (Rivera et al., 2005). As discussed earlier, transferrin (apotransferrin with bound Fe+3 ions) is critical for normal iron transport to cells. Liver synthesis and release of apotransferrin to plasma is increased in most species in response to iron deficiency (but not generally in dogs) and decreased in response to inflammation (negative acute phase protein). Transferrin deficient mice have iron deficiency anemia, but iron accumulates in the liver, presumably because of the ability of hepatocytes to take up NTBI (Verga Falzacappa and Muckenthaler, 2005). Ceruloplasmin is a glycoprotein with a molecular weight of 100 to 155 kDa depending on species. It migrates in the α2 region in humans but in the α1-region in horses on protein electrophoresis (Okumura et al., 1991). During biosynthesis, six atoms of copper are incorporated into ceruloplasmin late in the secretory pathway (Hellman and Gitlin, 2002). It contains most of the copper in the circulation in all species studied, except for the dog in which ceruloplasmin accounts for only about 40% of the plasma copper content (Montaser et al., 1992). It plays no role in copper transport or delivery to tissues (Hellman and Gitlin, 2002). Ceruloplasmin has ferroxidase enzyme activity that facilitates the oxidation of Fe+2 to Fe+3, a process involved in iron mobilization from liver and other tissue, but not from enterocytes (Osaki et al., 1971; Wessling-Resnick, 2006). It also appears to function as an antioxidant in plasma. Ceruloplasmin is a mild to moderate acute phase protein that increases in concentration in association with inflammation (Ceron et al., 2005; Smith and Cipriano, 1987). Its synthesis may also be enhanced by iron deficiency, estrogen, and progesterone. In contrast to iron, hepatocytes can excrete copper in the bile by a process that is dependent on the intracellular concentration of copper (Hellman and Gitlin, 2002). Haptoglobin is a glycoprotein of approximately 80 kDa molecular weight that contains approximately 20% carbohydrate. As an acute phase protein, the liver secretes increased amounts of haptoglobin into the circulation in response to inflammation (see Chapter 5). Plasma haptoglobin concentration may also increase following glucocorticoid administration in dogs and cattle (Harvey and West, 1987; Higuchi et al., 1994; Yoshino et al., 1993). Haptoglobin exists in dimer and polymer forms and is a major component of the α2-protein band identified by electrophoresis in most species. Lysis of erythrocytes in the circulation (intravascular hemolysis) releases free hemoglobin into plasma, and hemoglobin tetramers spontaneously dissociate into α–β dimers that are bound by haptoglobin. Each haptoglobin monomer can irreversibly bind a hemoglobin α–β dimer, preventing some hemoglobin loss (and therefore iron loss) in the urine following intravascular hemolysis. Macrophages remove hemoglobin-haptoglobin complexes from plasma, degrade the protein complex, and subsequently recycle the released iron. Haptoglobin also assists in the protection against bacterial infections by binding to free hemoglobin in infected tissues, limiting iron availability for bacterial growth. In addition, haptoglobin functions as an antioxidant because free hemoglobin promotes oxidative injury, which is inhibited by binding to haptoglobin (Melamed-Frank et al., 2001). Hepatocytes synthesize hemopexin, a 60-kDa acute phase plasma protein with a remarkably high binding affinity for heme. Free heme may be released in toxic amounts when intravascular hemolysis, rhabdomyolysis, or internal hemorrhage occur. Heme binds to cell membranes and other lipophilic structures, such as low-density lipoproteins, and promotes oxidative injury and inflammatory reactions. Binding of heme to hemopexin dampens these toxic effects. Like macrophages, hepatocytes can endocytose heme-hemopexin complexes following binding to CD91 on their surfaces. Once inside the cell, the complexes are transported to lysosomes for degradation and release of iron, and receptors are recycled to the cell surface (Hvidberg et al., 2005). When the hemoglobin concentration reaches a certain level in developing erythroid cells, it appears to signal the cessation of cell division. Abnormalities in heme or globin synthesis result in deficient hemoglobin synthesis and a delay in the signal for cell division to cease. When this happens, one or more extra cell divisions occur during erythroid cell development, resulting in the formation of microcytic erythrocytes (Stohlman et al., 1963). A deficiency in hemoglobin synthesis can also result in the formation of hypochromic erythrocytes with decreased hemoglobin concentration. Iron is essential for heme synthesis; consequently, iron deficiency results in deficient heme synthesis and the formation of microcytic, hypochromic erythrocytes. In addition to true iron deficiency, disorders that result in functional iron deficiency or defective iron incorporation into heme in mitochondria may result in the formation of microcytic, and possibly hypochromic, erythrocytes. These disorders include the anemia of inflammatory disease, copper deficiency, myelodysplastic disorders, drug or chemical toxicities, and possibly portosystemic shunts (Harvey, 2000). The mean cell volume (MCV) represents the average volume of a single erythrocyte in femtoliters (fl = 10–15 l). The MCV is determined most accurately with appropriately calibrated electronic cell counters that determine the size of individual cells and compute the MCV. The MCV varies greatly depending on species and, in some cases, depending on a breed within a species. Some dogs from Japanese breeds (Akita and Shiba) normally have MCV values below the reference intervals established for other breeds of dogs (Gookin et al., 1998), but these dogs are not anemic. The MCV is a fairly insensitive indicator of the formation of microcytes because a relatively high percentage of microcytes must generally be present in blood for the MCV to decrease below the reference interval. The mean cell hemoglobin concentration (MCHC) represents the average hemoglobin concentration within erythrocytes. It is calculated by dividing the hemoglobin value (in g/dl) by the hematocrit (as a percentage) and multiplying by 100. The MCHC is expressed as g/dl of erythrocytes. It may be normal in iron deficiency anemia when MCV values are only slightly decreased, but the MCHC is typically low when the MCV is moderately to markedly decreased. The mean cell hemoglobin (MCH) represents the average amount of hemoglobin in a single erythrocyte. It is calculated by dividing the Hb value (in g/dl) by the RBC count (in millions per μl) and multiplying by 10. The MCH generally provides little added information beyond that obtained from the MCV and MCHC because the MCH depends on both the size and hemoglobin concentration of erythrocytes. It usually correlates directly with the MCV, except in animals with macrocytic hypochromic erythrocytes. Exceptionally low MCH values strongly suggest true iron deficiency is present. Although quite useful when abnormal, MCV and MCHC values are relatively insensitive in identifying the presence of erythrocytes with abnormal volumes or hemoglobin concentrations. Many microcytic or macrocytic erythrocytes are required to move the MCV below or above the reference interval, respectively, and many hypochromic erythrocytes are needed to move the MCHC below the reference interval. In addition to counting cells, electronic cell counters can determine and plot the volume of individual erythrocytes, and examination of these erythrocyte volume histograms can reveal the presence of increased numbers of microcytes or macrocytes even when the MCV is within the reference interval (Weiser and Kociba, 1983). Some electronic cell counters such as the Advia 120 (Siemens Medical Solutions Diagnostics, Tarrytown, NY) can also determine the hemoglobin concentration of individual erythrocytes from the deflection of light that occurs when a laser beam strikes individual cells. Inspection of hemoglobin concentration histograms can reveal the presence of increased numbers of hypochromic erythrocytes, even when MCHC has not decreased below the reference interval. Individual erythrocytes can be further characterized by creating a cytogram in which the erythrocyte volumes of individual cells are plotted against their respective hemoglobin concentrations. In addition, the percentages of microcytes, macrocytes, hypochromic erythrocytes, hyperchromic erythrocytes, erythrocytes with low hemoglobin content, and erythrocytes with high hemoglobin content can be determined, and these same parameters can also be determined for reticulocytes in a blood sample. Because reticulocytes are recently formed immature erythrocytes, reticulocyte parameters may best reflect the current state of iron sufficiency in an animal. Alterations in several reticulocyte indices—including reticulocyte MCV (MCVretic), reticulocyte hemoglobin content (CHretic), percentage of hypochromic reticulocytes (% Hyporetic), percentage of reticulocytes with low hemoglobin content (% Low CHretic), percentage of reticulocytes with high hemoglobin content (% High CHretic), and percentage of macrocytic reticulocytes (% Macroretic)—appear to be of value in the diagnosis of iron deficiency in dogs (Fry and Kirk, 2006; Steinberg and Olver, 2005). Serum iron can be measured to assess the transport compartment of iron (Table 9-1). Serum iron concentration is increased in animals with hemolytic anemia and dyserythropoiesis, in which iron transfer from macrophages to plasma is increased (Harvey and Smith, 1994; Smith, 1992; Steffen et al., 1992; Stewart et al., 1953; Watanabe et al., 1998; Weiss and Lulich, 1999); in hypoplastic or aplastic anemia, in which iron transfer from plasma is decreased (Lange et al., 1976; Smith, 1992; Stokol and Blue, 1999; Stokol et al., 2000); in iron overload (Arnbjerg, 1981; House et al., 1994; Lavoie and Teuscher, 1993; Paglia et al., 2001; Sprague et al., 2003); in pigs with experimental pyridoxine deficiency (Deiss et al., 1966); in dogs and horses following the administration of glucocorticoid steroids (Fig. 9-7) (Adamama-Moraitou et al., 2005; Harvey et al., 1987b; Smith et al., 1986b); and in dogs with chronic hepatopathy (Soubasis et al., 2006). Serum iron concentrations are high at birth in foals, with most of the transferrin saturated with iron (Fig. 9-8), but serum iron decreases to adult values by 3 days of age (Harvey et al., 1987a). Although serum iron values in calves and piglets are not as high as values in foals at birth, a rapid decrease in serum iron also occurs in these species within a few days after birth (Kolb, 1963). Serum iron increases markedly in chickens associated with increased estrogen secretion and the onset of egg laying (Kolb, 1963). Serum iron values may be spuriously increased if laboratory tubes or pipettes used to handle serum are contaminated with iron. The use of disposable plastic pipettes and tubes minimizes this possibility. Iron concentrations may be spuriously increased in plasma samples if kits designed for serum iron determinations are used to measure iron in plasma.
I. INTRODUCTION
II. IRON DISTRIBUTION
A. Hemoglobin
B. Storage Iron
C. Myoglobin
D. Tissue Iron
E. Plasma Iron
III. IRON ABSORPTION
IV. PLASMA IRON TRANSPORT
V. REGULATION OF IRON METABOLISM
A. Intracellular Regulation of Iron Metabolism
1. Cytoplasmic Regulation of Iron
2. Mitochondrial Iron Metabolism and Regulation
B. Systemic Regulation of Iron Metabolism
VI. IRON METABOLISM IN CELLS
A. Iron Metabolism in Erythroid Cells
B. Iron Metabolism in Macrophages
C. Iron Metabolism in Hepatocytes
VII. TESTS FOR EVALUATING IRON METABOLISM
A. Hematology
B. Serum Iron
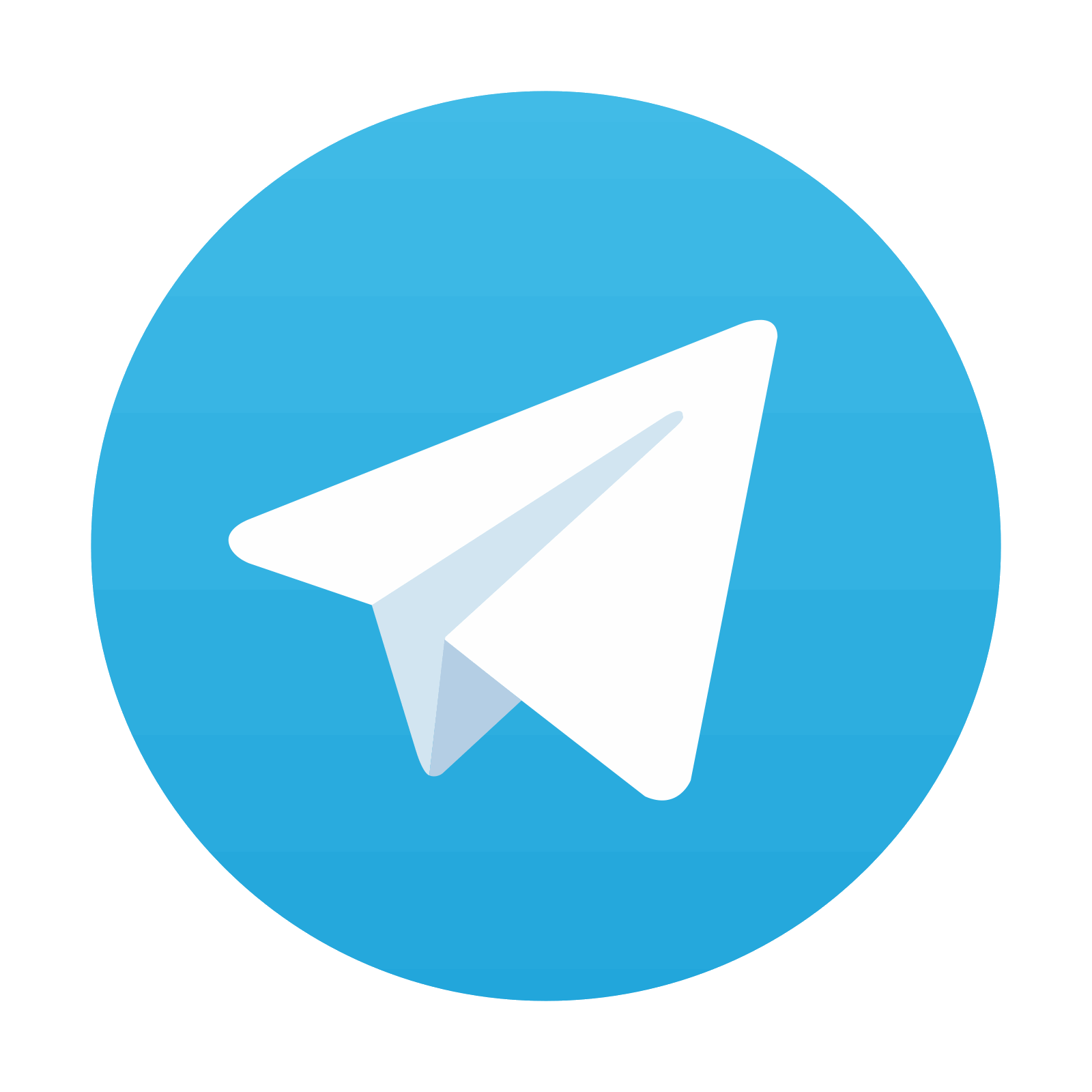
Stay updated, free articles. Join our Telegram channel
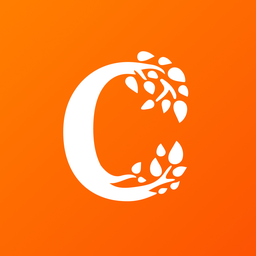
Full access? Get Clinical Tree
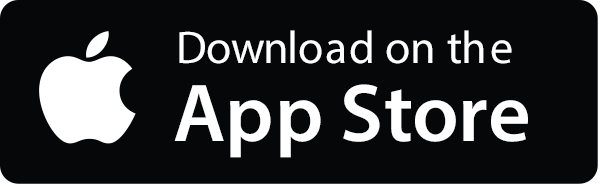
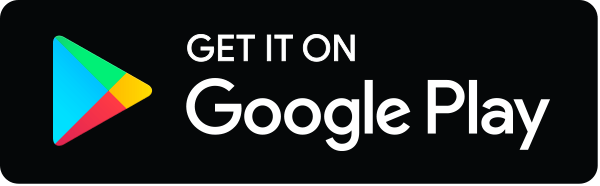