and Athina Markou1
(1)
Department of Psychiatry, School of Medicine, University of California San Diego, La Jolla, San Diego, CA, USA
Abstract
Intracranial self-stimulation (ICSS) is an operant behavioral paradigm in which experimental animals learn to deliver brief electrical pulses into specific regions of their own brains that are considered to be part of the brain’s reward pathways mediating both natural and ICSS reward. Several brain sites support ICSS, with the lateral hypothalamus, medial forebrain (MFB) bundle, and ventral tegmental area (VTA) among the sites that produce the most vigorous ICSS responding. Various ICSS procedures have been designed and used during the last decades since the discovery of ICSS. Two of the most commonly used ICSS procedures, which have been experimentally validated and have shown to be reward-selective, are the rate-frequency curve-shift procedure and the discrete-trial current-intensity threshold procedure. In all ICSS procedures, lowering of ICSS thresholds indicates a facilitation of brain stimulation reward, whereas elevations in ICSS thresholds reflect the diminished reward value of the stimulation and thus an anhedonic state. Acute administration of most drugs of abuse, including cocaine, amphetamine, nicotine, morphine, and heroin, lower ICSS thresholds in experimental animals. By contrast, withdrawal from chronic administration of these compounds induces elevations in ICSS thresholds, indicating an anhedonic state that resembles the negative affective state of the drug withdrawal syndrome experienced by humans. However, certain drugs of abuse, such as ethanol and cannabinoids, have shown inconsistent effects in ICSS procedures, primarily because of the minimal effects induced by these drugs in the ICSS procedure. In summary, the ICSS procedure provides unique ways to investigate the anatomical basis of reward and motivation and is an important tool for the assessment of the reward-facilitating and anhedonic effects of various drugs of abuse with addictive properties.
Key words
Intracranial self-stimulationRewardMotivationDrugs of abuseCocaineAmphetamineNicotineHeroinMorphineΔ9-TetrahydrocannabinolEthanolBenzodiazepinesPhencyclidineReward-facilitating effectAnhedoniaDrug dependenceDepression1 Introduction: History and Background of Brain Stimulation Reward
ICSS is an operant behavioral paradigm in which animals learn to deliver brief electrical pulses into specific parts of their own brain hypothesized to be part of the reward pathways that mediate both natural and ICSS reward (1–5). More than 50 years ago, Olds and Milner (1953) discovered that rats returned to an area of a test chamber where they had previously received electrical stimulation. Although the brain site stimulated in the original rat in this study is not specifically known, some believe it was the septum (6). This serendipitous observation of conditioned place preference to the electrical stimulus indicated that the stimulation was rewarding and led Olds and Milner to further train animals to perform an operant response to self-stimulate discrete brain areas (6). This discovery spurred major excitement in the field of reward and motivation. The field was provided with an important tool to study the brain sites and neuromechanisms involved in reward processes. The search for brain reward pathways has been shown to be far more complex than simply tracing the brain sites that would support ICSS. Nevertheless, ICSS, also termed brain stimulation reward, has been demonstrated to be a highly useful procedure in the delineation of the neurobiology of reward and motivation. It is worth mentioning that the terms ICSS and brain stimulation reward are distinct. Specifically, ICSS refers to an operational definition of the procedure in which a subject performs an operant response to stimulate parts of its brain, while the term brain stimulation reward has the explicit connotation that the stimulation is rewarding. Nevertheless, the two terms are often used interchangeably in the literature.
2 Basic Features and Parameters of the ICSS Procedure
Electrical stimulation usually consists of a 100–500 ms train of repeated pulses of sinusoidal or rectangular waveforms usually with a duration of 0.1 ms. The original studies of brain stimulation reward used a sinusoidal waveform, whereas the rectangular waveform is preferred more recently because it is hypothesized to stimulate neuronal fibers in a physiologically relevant manner (7, 8). The electrical stimulation can be anodal, cathodal, or of alternating polarity. Cathodal stimulation is more effective than anodal stimulation in exciting neurons (7). Furthermore, anodal stimulation stimulates neurons in a more complex way, so it is less preferred than cathodal stimulation (7).
The electrodes used in ICSS are either monopolar or bipolar. Bipolar electrodes deliver biphasic stimulation, meaning that the polarity around each electrode tip switches from pulse to pulse between anodal and cathodal stimulation, whereas monopolar electrodes deliver monophasic stimulation. Some monopolar electrodes have been designed to be moveable such that several brain sites may be explored in the same subjects along the dorsal–ventral axis (9). Moveable electrodes allow for changes in the location of the electrode tip and are mainly used in anatomical studies aimed at characterizing the sites supporting ICSS behavior (9). The effectiveness of a stimulating electrode depends on the size of the electrode tip, location of the electrode relative to the “reward” neurons and brain sites, current intensity, frequency, and train duration of the stimulation.
The most significant parameters of electrical brain stimulation are: (1) the current intensity (the maximal height of each pulse measured in μΑ); (2) the pulse width or duration of the stimulation pulses measured in milliseconds; (3) the frequency of the stimulation pulses that are a function of the width of each pulse and interpulse interval measured in Hz (i.e., number of pulses presented in 1 s), and (4) the train duration, measured in milliseconds, defined as the time period when pulses are delivered. Most recent studies use a pulse duration of 0.1 ms because it is considered the most physiologically relevant pulse duration that theoretically leads to one action potential (7). Any of the other three stimulation parameters – frequency (10), current-intensity (11), or train duration (12, 13) – can be varied to provide a reward threshold, whereas the other two parameters are kept constant. Such a threshold measure provides a quantitative assessment of stimulation efficacy and thus assesses brain reward pathway function. Different procedures are used to obtain and define reward thresholds (see below).
In most recent studies, either frequency or current intensity was chosen as the varied stimulation parameter. Changes in stimulation frequency are hypothesized to modify the firing frequency of the stimulated fibers, whereas changes in current-intensity modify the number of fibers that are activated around the stimulation tip (7, 14).
Lowering of ICSS threshold indicates an increase in the reward value of the stimulation because less electrical stimulation is required for the subject to perceive the stimulation as rewarding. Conversely, elevations in thresholds indicate a decrease in the reward value of the self-stimulation because higher frequencies or current-intensities are required before the subject perceives the stimulation as rewarding.
A number of different procedures have been developed and used in ICSS studies. Some of these procedures are the following: (1) rate-frequency (or rate-intensity or rate-duration) curve-shift procedure (12, 15–18), (2) discrete-trial current intensity procedure (11, 19, 20), (3) autotitration-of-threshold or set-reset procedure (21–24), (4) extinction or stimulus-control procedure (25–28), (5) post-reinforcement pause procedure (29, 30), (6) single-lever matching or response-strength procedure based on Herrnstein’s Matching Law (31, 32), and (7) one- and two-lever self-regulation of duration or On-Off procedure (33–35). However, not all of these procedures have been extensively used or experimentally validated. Furthermore, some of these procedures have limitations, the most important of which is that they are not reward-selective (36, 37). In some of these procedures, the simple response rate generated by a single arbitrary set of stimulation parameters is used, thus not providing a reliable measure of brain reward function (36, 37). The two procedures that have been the most extensively validated and used in most ICSS studies conducted to date are the rate-frequency curve-shift procedure (Fig. 1) and the discrete-trial current intensity procedure (Fig. 2). These two procedures appear to have the reward selectivity that is required in psychopharmacological research (14). Brief descriptions of each of these procedures are provided below, as well as a summary of the validation work conducted that has firmly established these two procedures in the ICSS field.
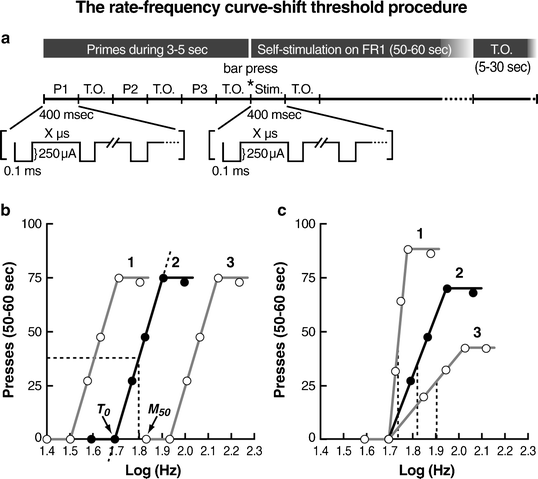
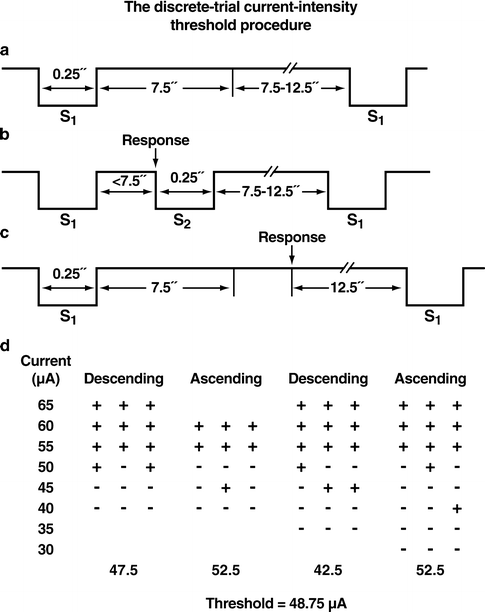
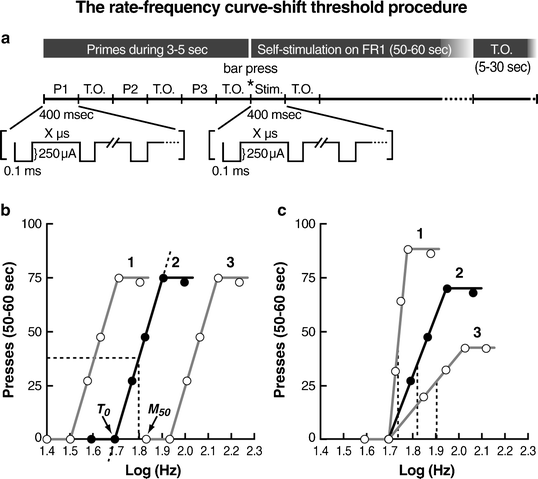
Fig. 1.
Rate-frequency curve-shift method of intracranial self-stimulation. (a) Schematic of representative stimulation parameters used in the rate-frequency curve-shift ICSS procedure. Typically, a trial begins with three stimulation primes (50 pulses/0.4 s/prime), with each prime consisting of a 0.4 s period of non-contingent stimulation administered at a constant current and frequency followed by a timeout (TO) period. The primes (P1, P2, P3) are typically administered for 3–5 s. When the animal presses the bar or nosepokes (the two most commonly used manipulanda with this procedure), stimulation (Stim) is delivered at the appropriate current and frequency for 0.4 s. Each cathodal pulse of current has a duration of 0.1 ms. The frequency determines how many pulses are delivered during this period, which varies from trial to trial. After each 50–60 s test period, a 5–30 s TO period occurs during which the animal can press the lever, but no additional stimulation is available. (b) Schematic depicting the theoretical functions that relate response rates (presses) to stimulation frequency (Log Hz). The rewarding efficacy of stimulation is measured by nonlinear regression in which a theoretical line (dashed line) is drawn through the curve at 60%, 50%, 40%, 30%, and 20% of the maximum rate of responding. Either the stimulation frequency that maintains half-maximal responding (Maximum 50, M 50; dashed line) or reflects the theoretical point at which the stimulation becomes rewarding (T 0; where dashed line 2 crosses the x-axis) can be used as the brain reward threshold. In this scenario, brain reward thresholds are either lowered (curve 1) (e.g., by increasing current or following acute administration of a drug of abuse) or elevated (curve 3) (e.g., by decreasing current or during withdrawal from a drug of abuse) compared with the control condition (curve 2). (c) Manipulations that affect response rates (e.g., decreasing (curve 1) or increasing (curve 3) the force required to depress the lever) can artificially affect M 50 brain reward thresholds. The M 50 (dashed lines) is a direct function of maximal response rates. By contrast, the T 0 (point of rise from x-axis) threshold measure is not affected by such motoric effects of manipulations because it is not a function of maximal response rates (Adapted from (39). With permission).
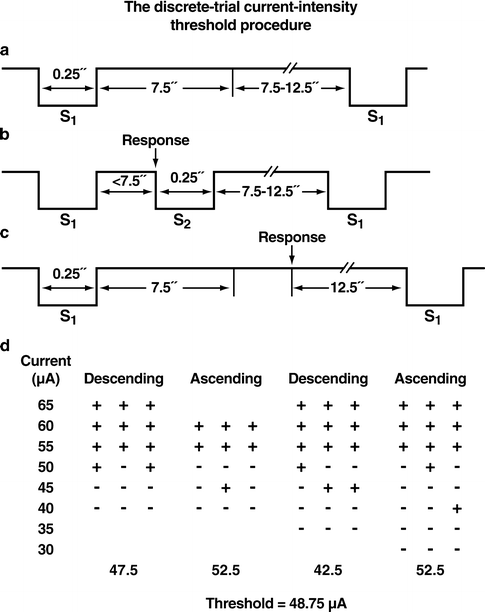
Fig. 2.
Discrete-trial current-intensity threshold intracranial self-stimulation procedure. Panels (a), (b), and (c) illustrate the timing of events during three hypothetical discrete trials. S1 refers to the non-contingent electrical stimulus that initiates the trial. S2 refers to the contingent electrical stimulus delivered after the completion of the operant response (see text for details). (a) A trial during which the animal did not respond (negative response). (b) A trial during which the animal responded within the 7.5 s response window (i.e., limited hold) after the delivery of the non-contingent stimulus (positive response). (c) A trial during which the animal responded during the inter-trial interval that resulted in the postponement of the initiation of the next trial by 12.5 s. The inter-response interval varies between 7.5 and 12.5 s. (d) A hypothetical session demonstrating how thresholds are defined for the four individual series of ascending and descending current intensities. The threshold of the session is the mean of the four series’ thresholds (Adapted from (11). With permission).
2.1 Rate-Frequency Curve-Shift Procedure
In the rate-frequency curve-shift procedure, the frequency of the stimulation is varied systematically, whereas the other parameters are held constant, so that the population of neurons that is stimulated is held constant. Animals typically press a lever manipulandum, nosepoke in a hole, turn a wheel manipulandum, or walk a runway to receive a train of stimulation. The completion of the operant response (e.g., lever-press) triggers a constant current generator to deliver a train of rectangular cathodal pulses of constant duration and intensity and variable frequency. The pulse frequency (i.e., number of pulses within a train) is progressively increased up to 40–50 pulses per stimulation train until the animal shows vigorous self-stimulation. As the frequency of the stimulation increases, the response rates rise accordingly until a maximal point of stimulation is delivered over which no further increases in response rates are produced and response rates reach an asymptote (15, 16). During the acquisition phase, the animals are trained to self-stimulate for at least 3 consecutive days, using stimulation parameters that maintain near maximal rates of responding. After the self-stimulation behavior has been acquired and stabilized for a given pulse frequency that supports maximal response rates, animals are trained to self-stimulate using four alternating series of ascending and descending pulse frequencies. The pulse frequency is varied by steps of approximately 0.1 log units. Each frequency is tested within trials of 50–60 s duration, followed by a timeout period of 5–30 s. These parameters can be varied between studies. At the beginning of each trial, the animals receive three to five trains of priming stimulation at the frequency of the stimulation that is available for that trial. The mean number of the four alternating ascending and descending pulse frequencies in each step leads to the generation of a sigmoidal frequency-response function, very similar to the dose-response function seen in pharmacological studies. A frequency-response function is established daily until the self-stimulation indices (i.e., threshold and asymptote measures) stabilize. Each of these daily sessions has a duration of approximately 40–50 min. Threshold and response rate asymptote measures are estimated based on the response function. Threshold is defined as the frequency, intensity, pulse width, or train duration that supports an arbitrary level of performance (see below for methods of estimation). The asymptote is the maximal response level exhibited by the subject. Thresholds reflect the rewarding efficacy of the stimulation, and response rate asymptotes are an estimate of an animal’s ability to perform the task and reflect motor or performance effects. Different quantitative measures are used in the rate-frequency curve-shift procedure to obtain threshold and asymptote (performance capability) estimates. The most reliable and commonly used measures are the M 50 (half-maximum) (15, 18), the Gompertz sigmoid growth model (which resembles the M 50) (38), and the Θ0 (39) (Fig. 1). The M 50 measure is analogous to the Effective Dose 50 (ED 50) used in pharmacology and refers to the stimulation frequency (or intensity or duration) that sustains responding at 50% of the maximal rate. The Θ0 measure refers to the theoretical point at which the stimulation becomes rewarding (i.e., response rates become higher than 0) (39). The M 50 measure can show artificial shifts attributable to increases or decreases in maximal response rates, whereas the Θ0 measure has been shown to be less affected by performance manipulations (8, 17, 18, 39). The Gompertz sigmoid growth model is a psychophysical method applied to the description of ICSS behavior:


In this equation, α represents the maximal rate (asymptote), and Xi (X at inflection) represents the threshold frequency. The latter is the pulse number producing 36.7% of the asymptotic rate (i.e., the rate lying on the fastest-accelerating region of the curve). Parameter b represents an index of the slope, and e is the base of natural logarithms. The advantage of this model compared with other estimates is that it accounts for every data point in the dose-response curve to measure the threshold and asymptote values (38).
Thus, this method enables distinguishing between reward and performance and quantifying the drug effect (8, 11, 18, 37, 39–41). The quantitative scaling of changes in reward is important for two reasons. First, the size of the reward-facilitating or reward-attenuating effect is useful when comparing the effects of different manipulations. Second, even small changes in brain stimulation reward or performance capability can be detected because a quantitative measure is less likely to have ceiling or floor effects (42). The same procedure may be used while varying the current-intensity (43) or train duration (12), generating rate-intensity or rate-duration curve functions, respectively, while keeping the other two parameters constant.
2.2 Discrete-Trial Current-Intensity Threshold Procedure
The discrete-trial current-intensity threshold procedure is a modification of a task initially developed by Kornetsky and Esposito (19). This procedure consists of discrete trials. Each trial begins with the delivery of a non-contingent electrical stimulus followed by a 7.5 s response window within which the subject can make a response to receive a second contingent stimulus identical to the initial non-contingent stimulus. Similar to all other ICSS procedures, this response may be a lever press, nose poke, or turning a wheel manipulandum, with the latter being the most commonly used response with this procedure. A response during this time window is a positive response, and the lack of a response is a negative response. Additional responses during a 2 s period immediately after a positive response have no consequence and are counted as extra responses. The inter-trial interval that follows either a positive response or the end of the response window (in the case of a negative response) has an average duration of 10 s (ranging from 7.5 to 12.5 s). Responses that occur during the inter-trial interval are timeout responses and result in a further 12.5 s delay of the onset of the next trial. During training on the discrete-trial procedure, the duration of the inter-trial interval and delay periods induced by timeout responses are gradually increased until animals perform consistently for a fixed stimulation intensity at standard test parameters. The animals are subsequently tested on the current-intensity threshold procedure in which stimulation intensities are varied according to the classical psychophysical method of limits (44). A test session consists of four alternating series of descending and ascending current intensities starting with a descending series (Fig. 2). Blocks of three to five trials are presented to the subject at a given stimulation intensity, and the current intensity is changed by steps of 5–10 μA between blocks of trials. The initial stimulus intensity is set approximately 40 μA above the baseline current-intensity threshold for each animal.
Each test session typically lasts 30–40 min and provides four dependent variables for behavioral assessment: threshold, response latency, extra responses, and timeout responses. The current-threshold for each descending series is defined as the stimulus intensity between the successful completion of a set of trials (i.e., positive responses during two or more of the three trials) and the stimulus intensity for the first set of trials, of two consecutive sets, during which the animal fails to respond positively on two or more of the three trials (in the case when three trials are presented at each current intensity). The current-threshold for each ascending series is defined as the stimulus intensity between a current intensity for which the animal fails to respond positively on two or more of the three trials and the first set of trials, of two consecutive sets, during which the animal responds positively on two or more of the three trials. The mean of the four series’ thresholds is defined as the threshold for the session. The time interval between the beginning of the non-contingent stimulus and a positive response is recorded as the response latency. The response latency for each session is defined as the mean response latency on all trials during which a positive response occurs. The extra responses and timeout responses for each test session are defined as the total number of extra responses and timeout responses that occur in the session, respectively. The response latency measure detects performance effects (11), extra responses primarily reflect the force with which a subject turns the wheel, and timeout responses reflect response inhibition (45). The main advantage of this procedure is that it allows for a rate-independent measurement of reward thresholds. Thresholds are defined independently of rate of responding which can be low because timeout responses result in postponement of the next trial (11). The discrete-trial current-intensity procedure has been used primarily with variations in current intensity, although other stimulation parameters can also be varied while keeping the current-intensity constant (8, 11).
In both of the above procedures (rate-frequency curve-shift and discrete-trial current-intensity threshold), data obtained for the threshold and response asymptote or response latency are usually expressed as a percentage of baseline to avoid the complexity of individual subjects having different baseline values for each of the measures (39).
3 Strengths and Weaknesses of the Intracranial Self-Stimulation Procedure
The ICSS procedure has several strengths and characteristics that distinguish it from other procedures used to study motivation, reward, or reinforcement, such as the self-administration and conditioned place preference paradigms. The ICSS procedure is considered a very powerful tool to assess whether a brain structure is involved in reward mechanisms because the electrode is implanted directly into the structure tested for brain stimulation reward (46). It is also a very flexible behavioral procedure that allows the implementation of different measurements. Furthermore, the ICSS paradigm offers one of the most direct measurements of drug effects on brain reward substrates (14, 46) (Table 1).
Table 1
Representative/selected articles on the effects of various drugs of abuse on ICSS thresholds
Drug | ICSS method | Species | Threshold lowering during acute/chronic drug administration | Threshold elevation during acute/chronic drug administration | Threshold elevation during drug withdrawal | No effect |
---|---|---|---|---|---|---|
Psychostimulants | ||||||
Cocaine | Continuous reinforcement schedule | Hooded rats | ↑ Response rates, 5 mg/kg, IP (125) | |||
Different stimulus parameter combinations | Wistar rats | ↑ Response rates, 0.6–10 mg/kg, SC (101) | ||||
Discrete-trial method | ||||||
Discrete-trial method | Albino male CDF rats | 2.5–20 mg/kg, IP (20) | ||||
Train-duration curve-shift method | Sprague-Dawley rats | 10–30 mg/kg, IP (103) | ||||
Variant current-intensity method | Wistar rats | 5 mg/kg, SC; 10, 30 mg/kg, IP (104) | ||||
Current-intensity method | Wistar rats | 5–20 mg/kg, IP (105) | 30, 40 mg/kg/twice daily for 18 days, IP (105) | |||
Train-duration method | Sprague-Dawley rats | ↑ Response rates, 15 mg/kg, IP (106) | ||||
Current-intensity method | Sprague-Dawley rats | ↑ Response rates, 5–30 mg/kg, IP (108) | ||||
Discrete-trial current-intensity method | Wistar rats | 2.5–20 mg/kg, IP (109) | ||||
Rate-intensity curve-shift method | Sprague-Dawley rats | 1–16 mg/kg, IP (110) | ||||
Rate-frequency curve-shift method | Sprague-Dawley rats | 5–30 mg/kg, IP (111) | ||||
Discrete-trial current-intensity method | Sprague-Dawley rats (males and females) | 1.8–18 mg/kg, IP (130) | ||||
Rate-frequency curve-shift method | Sprague-Dawley rats | |||||
Rate-frequency curve-shift method | Swiss-Webster mice | 2.5–20 mg/kg, IP (115) | ||||
Discrete-trial current-intensity method | Long-Evans rats | 4 mg/kg, IP (89) | ||||
Discrete-trial current-intensity method | Wistar rats | |||||
Rate frequency method | Long-Evans rats | 10 mg/kg, IP (118) | ||||
Rate frequency curve-shift method | Sprague-Dawley rats | 1.25–10 mg/kg, IP (120) | ||||
Rate frequency curve-shift method | Sprague-Dawley rats | 1.25–10 mg/kg, IP (123) | ||||
Rate frequency curve-shift method | Sprague-Dawley rats | 5 mg/kg, IP (124) | ||||
Current-intensity method | Wistar rats | 30 mg/kg/twice daily for 18 days, IP (105) | ||||
Rate-frequency curve-shift method | Sprague-Dawley rats | “Binge” cocaine, 15 mg/kg/injection, IP, for 7 days (133) | ||||
Train-duration method | Sprague-Dawley rats | 10–15 mg/kg for 18 days, IP (103); ↑ response rates, 15 mg/kg, IP (126) | ↓ Response rates, 25 mg/kg for 18 days or 30 mg/kg for 3 days, IP (126) | |||
Drug | ICSS method | Species | Threshold lowering during acute/chronic drug administration | Threshold elevation during acute/chronic drug administration | Threshold elevation during drug withdrawal | No effect |
Discrete-trial current-intensity method | Wistar rats | 8 Injections of 15 mg/kg over 9 h, IP (134) | ||||
Discrete-trial current-intensity method | Wistar rats | |||||
D-Amphetamine | Rate-intensity method | BALB/c, DBA/2 and C57BL/6 mice | ↑ Response rates, 0.25–8 mg/kg, IP (157) | |||
Rate-intensity method | Sprague-Dawley rats | ↑ Response rates, 0.25–2 mg/kg, IP (158) | ||||
Rate-dependent various intensities method | Long-Evans rats | ↑ Response rates, 0.5–2 mg/kg, IP (156) | ||||
Continuous reinforcement schedule | Long-Evans hooded rats, albino Walter Reed rats | ↑ Response rates, 0.5–5 mg/kg, IP (154) | ||||
Rate-intensity method | Sprague-Dawley rats | ↑ Response rates, 0.1 mg/kg, SC (155) | ||||
Rate-intensity method | Wistar rats | ↑ Response rates, 1.5 mg/kg for 9 days, IP (152) | ||||
Photobeam modified hole-board task | Swiss mice | ↑ Response rates, 0.5, 1, and 2 mg/kg, IP (51) | ↓ Response rates, 3 and 5 mg/kg, IP (51) | |||
Rate-intensity method | Charles River albino rats | 2 and 4 mg/kg, IP (96) | ||||
Autotitration and discrete-trial threshold detection method | Sprague-Dawley rats | 0.03–1 mg/kg, IP (138) | ||||
Variant train duration method | Sprague-Dawley rats | 0.33, 0.66, and 1 mg/kg counterbalanced for 3 days, IP (126) | ||||
Discrete-trial current-intensity method | Wistar rats | 1–6 mg/kg for 2 days, IP (143) | ||||
Rate-frequency curve-shift method | Sprague-Dawley rats | 1 μg/side into the nucleus accumbens shell (141) | ||||
Rate-frequency curve-shift method | Dopamine D2 receptor wildtype heterozygous, and knockout mice | 1–4 mg/kg, IP (139) | ||||
Discrete-trial current-intensity method | Wistar rats | 5 and 10 mg/kg/day for 7 days, SC (163) | 5 and 10 mg/kg/day for 7 days, SC (163) | |||
Continuous reinforcement schedule | Hooded rats | 2.5–10 mg/kg 3 times daily for 5 days, IP (171) | ||||
Decreased current-intensity method | Sprague-Dawley rats | 5 mg/kg for 7 days followed by 10 mg/kg for 7 days, IP (160) | ||||
Response rates in 10 min sessions | Swiss mice | 7.5 mg/kg twice daily for 10 days, IP (151) | ||||
Drug | ICSS method | Species | Threshold lowering during acute/chronic drug administration | Threshold elevation during acute/chronic drug administration | Threshold elevation during drug withdrawal | No effect |
Photobeam task of ICSS | Male albino rats | 7.5 mg/kg twice daily for 10 days, IP (161) | ||||
Rate-intensity discrimination method | Wistar rats | 10 mg/kg for 12 days, IP (162) | ||||
Rate-frequency curve-shift method | Long-Evans rats | 1–10 mg/kg, IP, twice daily for 5 days per week for 6 weeks (168) | ||||
Discrete-trial current-intensity method | Wistar rats | |||||
Discrete-trial current-intensity method | Wistar rats | |||||
Nicotine | Photobeam current-intensity ICSS task | Lister hooded rats | 0.4 mg/kg, SC (177) | |||
Continuous reinforcement schedule and autotitration method | Sprague-Dawley rats | 0.03–1 mg/kg, SC (52) | ↓ Response rates, 0.03–1 mg/kg, SC (52) | 0.03–1 mg/kg, SC (52) | ||
Fixed-ratio 15 schedule | Sprague-Dawley rats | ↑ Response rates, 0.1 mg/kg, SC (52) | ↓ Response rates, 1 mg/kg, SC (52) | |||
Different-schedules methods | Sprague-Dawley rats | ↑ Response rates, 0.01–0.3 mg/kg, SC (188) | ||||
Autotitration method | Wistar rats | 1 mg/kg, SC (180) | ||||
Progressive-ratio method | Wistar rats | 1 mg/kg, SC (180) | ||||
Discrete-trial current-intensity method | Wistar rats | 0.125–0.5 mg/kg, SC (50) | ||||
Discrete-trial current-intensity method | Wistar rats | 0.03 mg/kg/infusion for 1 h or 12 h daily (48) | ||||
Rate-frequency curve-shift method | Sprague-Dawley rats | 0.5 mg/kg, SC (114) | ||||
Discrete-trial current-intensity method | Wistar rats | |||||
Discrete-trial current-intensity method | Wistar rats | 3.16 mg/kg/day for 7 days, SC (55) | ||||
Discrete-trial current-intensity method | Wistar rats | |||||
Discrete-trial current-intensity method | Wistar rats | Precipitated withdrawal, 9 mg/kg/day salt for 14 days, SC (195) | ||||
Discrete-trial current-intensity method | Wistar rats | Precipitated withdrawal, 3.16 mg/kg/day for 14 days, SC (209) | ||||
Drug | ICSS method | Species | Threshold lowering during acute/chronic drug administration | Threshold elevation during acute/chronic drug administration | Threshold elevation during drug withdrawal | No effect |
Discrete-trial current-intensity method | Wistar rats | |||||
Discrete-trial current-intensity method | Wistar rats | Precipitated withdrawal, 9 mg/kg/day salt for 14 days, SC (204) | ||||
Discrete-trial current-intensity method | Wistar rats | Precipitated withdrawal, 3.16 mg/kg/day for 14 or 28 days, SC (218) | ||||
Discrete-trial current-intensity method | Wistar rats | 3.16 mg/kg/day for 7 days, SC (205) | ||||
Discrete-trial current-intensity method | C57BL6 mice | Spontaneous and precipitated withdrawal, 2 mg/kg/injection 4 times daily for 7 days, SC, or 24 mg/kg for 13 days, SC (196) | ||||
Discrete-trial current-intensity method | Wistar rats | 3.16 mg/kg/day for 7 and 28 days, SC (183) | ||||
Discrete-trial current-intensity method | Wistar rats | Precipitated withdrawal, 3.16 mg/kg/day for 28 days, SC (208) | ||||
Discrete-trial current-intensity method | C57BL/6J mice | 40 mg/kg/day for 28 days, SC (198) | ||||
Opiates | ||||||
Morphine | Discrete-trial method | CDF rats | 4–12 mg/kg twice daily for up to 25 days, IP (226) | |||
Fixed-interval method | Sprague-Dawley rats | ↓ Response rates, 3 and 5.6 mg/kg, IP (238) | ||||
Discrete-trial method | Fischer-344 rats | 0.015–0.5 mg/kg, SC (230) | ||||
Autotitration and progressive ratio method | Wistar rats | 1–10 mg/kg, SC (180) | ||||
Rate-frequency curve-shift method | Long-Evans rats | Morphine-associated cues, 5 mg/kg, IP (118) | ||||
Autotitration method | Sprague-Dawley rats | |||||
Drug | ICSS method | Species | Threshold lowering during acute/chronic drug administration | Threshold elevation during acute/chronic drug administration | Threshold elevation during drug withdrawal | No effect |
Progressive-ratio schedule of ICSS | Sprague-Dawley rats | Naltrexone-precipitated withdrawal, 3 and 5.6 mg/kg, SC (244) | ||||
Discrete-trial current-intensity method | Wistar rats | 0.01–0.03 mg/kg, SC, naloxone-precipitated withdrawal, dependent rats (morphine 3–10 days) (246) | ||||
Discrete-trial current-intensity method | Wistar rats | Precipitated withdrawal, 75 mg morphine SC pellets for 7 days (209) | ||||
Heroin | Rate current-intensity method | Albino, Wistar-derived rats | ↑ Response rates, 5 mg/kg for 5 days, IP (239) | |||
Fixed-ratio 1 schedule | Sprague-Dawley rats | ↑ Response rates, 10–300 μg/kg/injection, self-administered (240) | ||||
Discrete-trial current-intensity method | Wistar rats | Non-dependent rats, 30 μg/kg, SC (57) | Naloxone-precipitated withdrawal, 30 μg/kg, SC, dependent rats (heroin 1 h and 23 h sessions) (57) | |||
Discrete-trial method | Fischer-344 rats | 0.06 and 0.5 mg/kg, SC (230) | ||||
Cannabinoids | ||||||
Δ9-THC | Current intensity autotitration method | Lewis rats | ||||
Rate-frequency curve-shift method | Lewis and Sprague-Dawley | 1 mg/kg, IP (252) | ||||
Rate-frequency curve-shift method | Fischer-344 rats | 1 mg/kg, IP (252) | ||||
Current intensity method | Long-Evans rats | 0.12–10 mg/kg, PO (255) | ||||
Levonantradol | Discrete-trial current-intensity method | Albino CDF rats | 0.2, 0.3 mg/kg, SC (256) | |||
Rate-frequency curve-shift method | Sprague-Dawley rats | 1 and 2 mg/kg, IP (257) | ||||
PCP | Fixed interval schedule | Sprague-Dawley rats | 3 mg/kg (238) | 5, 10 mg/kg, SC, acutely and chronically for 14 days (56) | ||
Drug | ICSS method | Species | Threshold lowering during acute/chronic drug administration | Threshold elevation during acute/chronic drug administration | Threshold elevation during drug withdrawal | No effect |
Current intensity autotitration or progressive-ratio schedule | Wistar rats | 0.3–5.6 mg/kg (180) | ||||
Rate-frequency curve-shift method | Long-Evans rats | |||||
MDMA | ||||||
Rate-independent discrete-trial method | F-344 rats | 2 mg/kg, SC (295) | ||||
Variable three-intensity method | Sprague-Dawley rats | ↑ rates of pressing, 2 mg/kg, SC 296 | ||||
Ethanol | ||||||
Current intensity autotitration method | Sprague-Dawley rats | ↓ Lever presses, 5% ethanol-containing liquid diet (275) | 1.7 g/kg, IP or IG (274) | |||
Rate-intensity ascending threshold schedule | Holtzman albino rats | 0.9 and 1.2 g/kg,IP (272) | 0.6 g/kg, IP (272) | |||
Current intensity autotitration method | Wistar rats | 0.6–2.4 g/kg, PO (180) | ||||
Discrete-trial current-intensity method | Wistar rats | ↑ Threshold, 22 mg/liter ethanol vapor for 17–20 days (54) | ||||
Rate-frequency method | High and low alcohol drinking N/NIH outbred rats | ↑ Threshold and ↓ lever presses in the low alcohol drinking group, 4 g/kg, IG, once (276) |
One of the most obvious strengths of brain stimulation reward is that it is a rapidly acquired response because of the powerful rewarding effects of ICSS and the fact that no delay occurs between the animal’s operant response and the delivery of the stimulation. Animals that respond for ICSS will choose to press a lever or turn a wheel to self-administer electrical stimulation over natural rewards, such as food and water, or in a subfreezing environment for hours or even days to the point of exhaustion (47). Moreover, ICSS directly activates the brain reward systems that drive behavior, bypassing most of the input side of these neuronal circuits (e.g., bypassing taste buds and their signal to the brain). No other behavior related to reward consumption can interfere with the operant response or complicate data interpretation.
Another advantage of ICSS procedures is that they provide quantitative measures of brain reward function and the effects of manipulations. Notably, however, because of the wide variety of ICSS procedures and stimulation parameters, direct comparisons of the magnitude of reward-enhancing or reward-attenuating effects are sometimes difficult to be determined between studies. Another advantage of the ICSS procedure is that all parameters of brain stimulation reward can be controlled and measured more precisely than those of natural rewards. Furthermore, the effects of various manipulations can be assessed using ICSS of different areas of the brain, potentially providing insights into the neurosubstrates of the effects of specific manipulations.
Another strength of the ICSS paradigm is that in almost all currently used ICSS procedures one can readily distinguish between the reward-enhancing (threshold) and motor/performance effects (response latency or maximal response rate) of manipulations. The ICSS procedure can be used to test either the acute or chronic effects of a drug, or even both (Table 1). Repeated testing, even several times daily, is feasible because no satiation occurs to the rewarding effects of the stimulation, thus allowing for detailed time-course assessments. Provided that the surgeries are conducted carefully to precisely position the electrode in the intended brain site and the head mount is well-secured to the skull, subjects can be tested routinely for periods of months or up to 1 year. During that time, unless manipulations are implemented, reward thresholds are extremely stable. Finally, the ICSS procedure does not require deprivation of any kind (e.g., food or water deprivation), which is the case for other reinforcers, for the animals to learn to perform reliably in these procedures.
In drug abuse research, the ICSS procedure provides a means of quantifying the reward-enhancing or reward-facilitating effects of drugs of abuse (48–50). ICSS procedures also allow the assessment of the reward deficits characterizing withdrawal from chronic exposure to drugs of abuse, reflected in elevated brain reward thresholds (51–57). In summary, the use of the ICSS procedure provides a unique way of studying the neurobiology of reward and motivational processes and provides quantitative measures of brain reward function and changes in brain reward function.
Nevertheless, the ICSS procedure also has some limitations. One of its limitations is the seemingly artificial nature of the reward. The procedure involves direct activation of brain reward circuits, bypassing the input side of the system. Although this is also an advantage of the procedure, researchers outside of the field may not readily know what the behavior is and what exactly is measured, thus sometimes making the communication of findings difficult. Another disadvantage of the procedure is that it requires surgery which can be labor-intensive. Nevertheless, this disadvantage is offset by the fact that the procedure can be used to provide multiple data points, including detailed time-course analyses. Finally, another disadvantage is that an electrode has to be implanted and secured to the skull, thus rendering the implementation of other brain manipulations, such as intracerebral injections, more difficult. Both the electrode and access to another brain device, such as an intracerebral cannula, need to be provided on the same small skull surface.
4 Brain Sites and Systems That Support Rewarding Intracranial Self-Stimulation
The brain pathways activated by ICSS are hypothesized to be the same pathways that are activated by natural rewards and by drugs of abuse that exert a powerful influence on pathways involved in incentive-motivation (1–4). Olds (58) and subsequent workers in the field (59–61) hypothesized that the posterior MFB region of the lateral hypothalamus was a major component of the brain reward system. At that time, when most researchers believed that the brain had only one unitary reward system, Stein proposed the noradrenergic hypothesis of brain stimulation reward, based on neuroanatomical and neurochemical evidence indicating a selective role of norepinephrine in the locus coeruleus (62, 63). However, subsequent neurochemical, mapping, lesion, and autoradiographic studies (64) showed that the brain reward system comprises several interconnected subsystems responsible for the rewarding effects of natural reinforcers and the reward-enhancing effects of addictive drugs (19, 65). Several brain sites will support brain stimulation reward, including the MFB region of the hypothalamus and especially the lateral and posterior part of the hypothalamus and regions of the mesolimbic dopamine system, the substantia nigra pars compacta, VTA (66), central nucleus of the amygdala (67), septum (6), bed nucleus of the stria terminalis, and nucleus accumbens (NAcc). The raphe nuclei (68) and different sites of the frontal and prefrontal cortex (PFC) (for review, see (69)) are also part of the brain reward system and support ICSS behavior. Many studies demonstrated that the medial PFC is a brain region highly implicated in ICSS behavior (70–73). Additional structures that support ICSS behavior include the hippocampus (74), amygdala, locus coeruleus (75, 76), caudate nucleus, olfactory bulb, tubercle (77), and cerebellum (78–80).
Notably, however, each of these structures that support ICSS cannot be considered a self-contained reward system that can generate rewarding effects as part of a unique reward system. The MFB, for example, has not just one unitary and exclusive reward system located in the MFB; multiple, parallel, and at times interdependent and interconnected reward systems may be located in different brain regions to mediate reward processes (65, 81). Although all of these structures were initially hypothesized to be part of the brain reward system and to be activated by ICSS to produce rewarding effects, extensive studies demonstrated that reward is not a unitary process, but rather a complex process composed of various and sometimes different psychological processes and underlying brain mechanisms (82–84).
5 Effects of Major Drugs of Abuse on Intracranial Self-Stimulation
5.1 Psychomotor Stimulant Drugs
Psychomotor stimulants, such as amphetamine, cocaine, and methamphetamine, are monoamine reuptake inhibitors or releasers, which exert their effects by activating mainly the dopaminergic, noradrenergic, serotonergic, and glutamatergic neurotransmitter systems of the brain (85–89). These brain systems are localized, among others, in brain regions highly implicated in brain reward functions, such as the NAcc, VTA, and PFC (90, 91).
Amphetamines and cocaine potentiate brain stimulation reward when they are administered either intracranially or systemically (3, 19, 20, 22, 35, 51, 53, 92–100). Specifically, a plethora of studies have shown that acute cocaine administration potentiates ICSS behavior reflected in increased response rates and lowering of ICSS thresholds in rats and mice (Fig. 3), whereas during cocaine withdrawal, decreased response rates and elevated ICSS thresholds were observed (19, 20, 89, 93, 101–128) (Fig. 4). Using different ICSS methods and stimulus parameters, acute administration of a variety of cocaine doses, ranging from 0.6 to 20 mg/kg, dose-dependently increased response rates (101) or lowered ICSS thresholds (19, 20, 93). Extending these findings, Markou and colleagues reported that repeated cocaine administration (10 mg/kg for 20 consecutive days) lowered ICSS thresholds, with no tolerance or sensitization to this effect (116). This latter procedure led to classical conditioning of the reward-facilitating effects of cocaine, such that after cessation of cocaine administration, a conditioned lowering of reward thresholds was observed upon re-exposure to the same testing conditions and following a saline injection (116). Kelley and Hodge shed more light on the reward-facilitating effects of cocaine by showing that these effects are mediated through 5-HT3 receptor activity (89). Other research groups demonstrated that other systems in the brain, such as hypocretin, γ-aminobutyric acid, and cannabinoid, may also affect the reward-facilitating effects of cocaine (119, 121, 123, 124). Interestingly, using the discrete-trial current-intensity method, Markou and colleagues found that self-administration of low doses of cocaine (10 and 20 injections of 0.25 mg/kg) lowered reward thresholds, an effect that only lasted for a short time (less than 2 h). Higher doses and testing in the ICSS procedure at later time points revealed no reward-enhancing effect of cocaine (129). These effects of self-administered cocaine were similar to those seen after intraperitoneal administration of 10 mg/kg cocaine (see above) and did not differ between male and female rats (130).
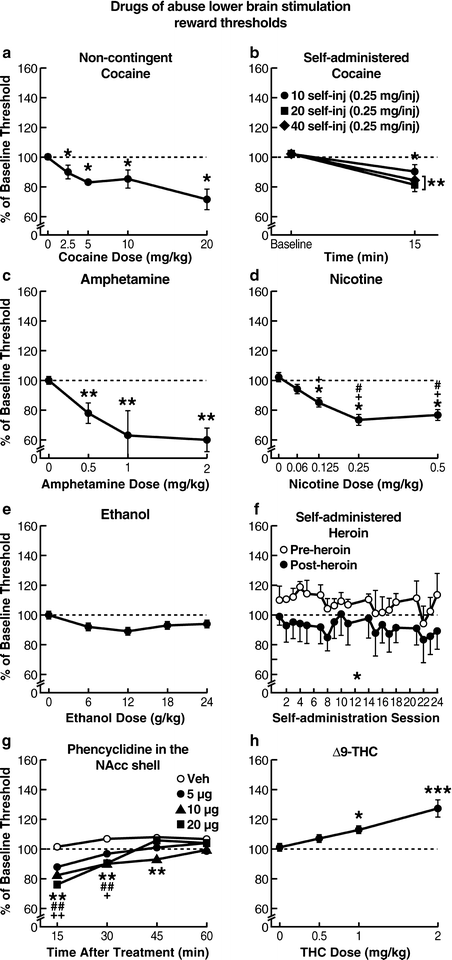
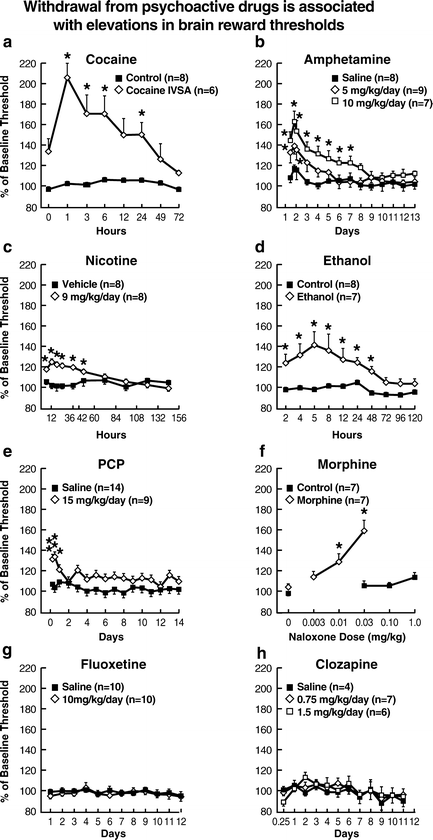
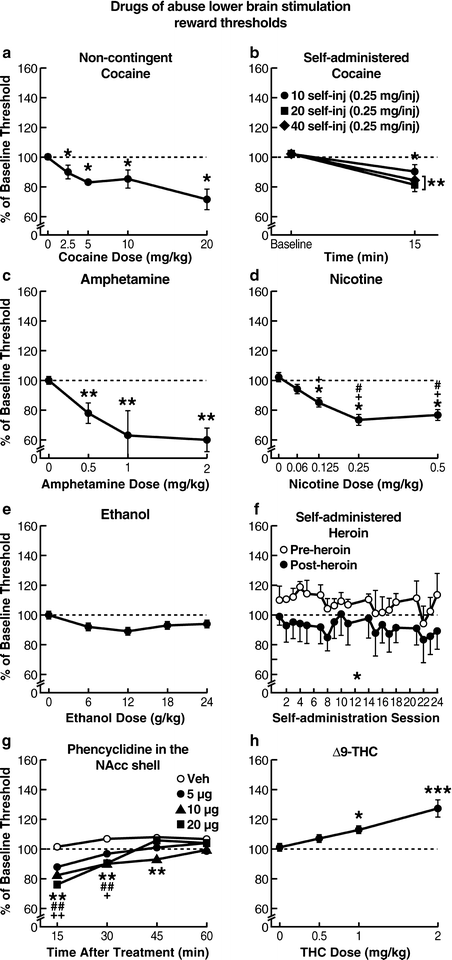
Fig. 3.
Drugs of abuse lower brain stimulation reward thresholds. Administration of a wide variety of psychoactive drugs leads to potentiation of activation of brain reward pathways activated by ICSS, reflecting a reward-facilitating effect of these compounds. Administration of drugs that are highly rewarding to humans, such as cocaine, amphetamine, nicotine, ethanol, heroin, and phencyclidine, all lower ICSS thresholds, indicating a reward-facilitating effect. By contrast, drugs that show a biphasic effect in humans, such as Δ9-THC, do not show a reward-enhancing effect in the ICSS procedure. Notice that direct comparisons of effect magnitudes cannot be made among different drugs in these figures because different ICSS procedures and parameters were used to generate the depicted findings (a and b: Adapted from (11, 116), respectively; c: Adapted from (297); d: Adapted from (50); e: Adapted from (180); f: Adapted from (48); g: Adapted from (290); h: Adapted from (257). With permission).
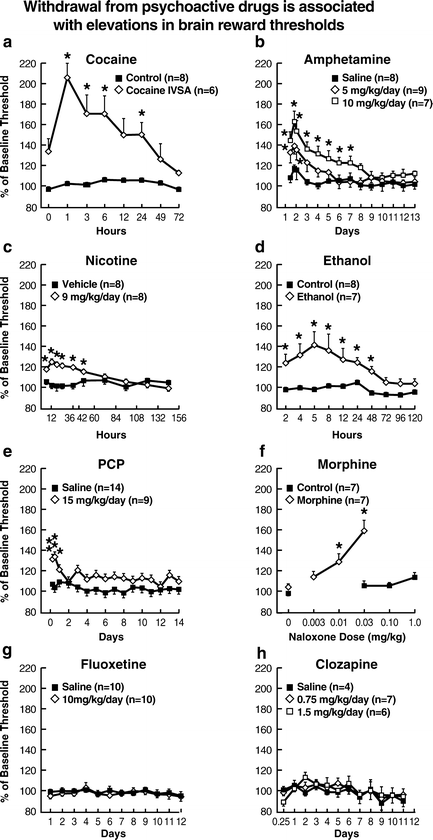
Fig. 4.
Withdrawal from psychoactive drugs is associated with elevations in brain reward thresholds. Withdrawal from a wide range of psychoactive drugs induces robust deficits in brain reward function. These affective sequelae can be quantified with the ICSS procedure. When responding for ICSS from electrodes, anhedonia is operationally defined as elevations in ICSS thresholds (either current-intensity, frequency, or train-duration thresholds). Withdrawal from drugs that are rewarding to humans, such as psychostimulants (cocaine (a), amphetamine (b), and nicotine (c)), depressants (ethanol (d) and morphine (f)), and an NMDA receptor antagonist (phencyclidine (e)), all elevated reward thresholds. By contrast, drugs that are not rewarding to humans, such as fluoxetine and clozapine (g) and (h), did not alter reward thresholds after termination of chronic administration, thereby demonstrating the discriminant validity of the paradigm. Notice that direct comparisons of effect magnitudes cannot be made among all figures because different stimulation parameters and procedures were used to conduct the cocaine, morphine, and ethanol studies (a–c: From (53, 164); d, f: From (54, 246); e: From (56); g, h: From (173, 296). With permission).
Other studies using the rate-intensity or rate-frequency curve-shift methods also showed that cocaine leads to facilitation of brain stimulation reward, indicated by lowering of ICSS thresholds (110, 111, 115). Frank and colleagues (103) and van Wolfswinkel and colleagues (104) used even higher doses of cocaine (5–30 mg/kg, IP or SC) and tested the animals in a varied train duration or current-intensity ICSS procedure, respectively, with the ICSS electrode implanted at the level of the VTA. Cocaine significantly lowered train duration thresholds, again demonstrating a reward-facilitating effect of cocaine regardless of the ICSS method used (103, 104). Similar effects were also seen after acute or subchronic administration of cocaine when the electrode was located in the ventral pallidum (112), PFC (106), or medial PFC (107) or when the operant response varied (i.e., nosepoking or lever-pressing) in a rate-dependent ICSS procedure (108). Specifically, cocaine (1.5–10 mg/kg, IP) dose-dependently lowered ICSS thresholds of the ventral pallidum or lateral hypothalamus in the rate-frequency curve-shift procedure (112, 114), and this effect was not as pronounced when the animals were treated with methylphenidate during early developmental stages (120).
Using the rate-frequency curve-shift ICSS procedure in mice, acute cocaine administration (2.5–20 mg/kg, IP) dose-dependently lowered brain stimulation reward thresholds (115), whereas repeated administration of high-dose cocaine (40 mg/kg) decreased response rates and elevated thresholds (105). Consistent with one of the advantages of ICSS is the finding that these effects of cocaine are independent of the psychomotor stimulant effects of cocaine. Doses that induce enhancement of brain stimulation reward do not lead to significant effects on the performance capability of the animals (111, 112).
During cocaine withdrawal, the impact of rewarding brain stimulation is attenuated, quantified by elevations in ICSS thresholds, indicating a depression-like state (53, 105, 109, 131–135) (Fig. 4). In a rate-intensity ICSS procedure, rats received repeated administration of a high cocaine dose (40 mg/kg, IP) immediately after each daily ICSS session for 7 days and were tested 24 h later (105). This repeated cocaine administration led to decreased response rates and elevated thresholds in the ICSS testing of each subsequent day (24 h post-injection). Repeated cocaine administration (30 mg/kg, twice daily) for 18 days led to lowering of thresholds and decreases in response rates when animals were tested for ICSS 5 days after discontinuation of cocaine administration (105). Importantly, in studies by Markou and Koob, in which the discrete-trial current-threshold ICSS procedure was used and cocaine (0.5 mg/kg/injection) was intravenously self-administered by rats under a fixed-ratio 5 schedule of reinforcement for 3–72 h continuously, thresholds were elevated for up to 72 h post-cocaine self-administration for the longest cocaine exposure (53, 109, 131). This anhedonic effect of cocaine withdrawal has been shown to be associated with decreased dopaminergic (109, 136), decreased noradrenergic (131), and increased adenosine (134, 135) neurotransmission. Furthermore, Markou and colleagues showed that repeated administration of the tricyclic antidepressant desmethylimipramine (DMI) (10 mg/kg, IP, twice daily for 5 days) significantly downregulated β-adrenergic receptors and shortened the duration of post-cocaine anhedonia reflected in reversal of the threshold elevations 1, 3, and 6 h after the termination of a 12 h cocaine self-administration session (0.5 mg/kg/injection) in the discrete-trial current-threshold ICSS procedure (131). In this study, the level of β-adrenergic receptor downregulation correlated significantly with the degree of effectiveness of DMI treatment in reversing post-cocaine anhedonia (131). Baldo and colleagues showed that repeated administration of cocaine (15 mg/kg, IP, eight injections over 9 h) produced elevations in thresholds 4, 8, and 12 h after cocaine administration, and this effect was reversed by administration of the adenosine-2 (A2) receptor-selective antagonist 3,7-dimethyl-1-propargylxanthine (DMPX) (3 and 10 mg/kg) administered before both the 8 and 12 h post-cocaine self-stimulation testing sessions (134). Goussakov and colleagues recently showed using the rate-frequency curve-shift ICSS procedure that cocaine withdrawal attenuated the impact of rewarding brain stimulation, reflected in elevated ICSS thresholds, an effect that was evident after 3 and 5 days of a 7-day regimen of “binge” cocaine treatment (15 mg/kg/injection, IP, 3 daily injections at 1 h intervals) (133).
Similar to cocaine, D-amphetamine administration induces reward-enhancing effects in the ICSS procedure in rats, with electrodes located in the lateral hypothalamus, dorsal noradrenergic bundle, PFC, substantia nigra, and VTA (22, 96, 137–159) (Fig. 3). Withdrawal from D-amphetamine results in elevations in ICSS reward thresholds (51, 160–172) (Fig. 4).
Cazala assessed the effects of D- and L-amphetamine in three different mouse strains with electrodes implanted in dorsal and ventral hypothalamic areas (0.25–8 mg/kg, IP) and found that both isomers increased self-stimulation response rates in all three strains, although with different sensitivity for each mouse strain (157). Using a modified hole-board task for self-stimulation in mice, Kokkinidis and Zacharko found that low D-amphetamine doses increased, and high doses decreased, ICSS response rates (51). A recent study by Elmer and colleagues, using the rate-frequency ICSS procedure in dopamine D2 receptor deficient mice, showed that amphetamine (1–4 mg/kg, IP), in contrast to morphine, potentiated brain stimulation reward across the three genotypes used in the study (D2 wildtype, heterozygous, and knockout mice), indicated by equal leftward shifts in the rate-frequency functions (139).
D-amphetamine appears to elicit differential anatomical sensitivity, measured by selective increases in response rates for ICSS on a fixed-ratio 1 schedule of reinforcement in rats prepared with electrodes in various brain sites (154). Specifically, rats prepared with electrodes in the septal area, the anterior and posterior hypothalamus (0.62, 2.5, and 5 mg/kg), or the ventromedial tegmentum and locus coeruleus (0.5–4 mg/kg) exhibited increased responding after D-amphetamine administration, with the highest responding when electrodes were implanted in the ventromedial tegmentum and posterior lateral hypothalamus (154). Another study showed that a low amphetamine dose (0.1 mg/kg) induced higher facilitation of brain stimulation reward when the electrode was placed more dorsally or medially in the substantia nigra than when it was placed close to the dorsal border of the substantia nigra (155). Additionally, self-stimulation of the PFC was facilitated by chronic D-amphetamine administration (1.5 mg/kg for 9 days), indicated by increases in response rates, whereas self-stimulation of the NAcc and supracallosal bundle remained unchanged or was suppressed, respectively (152).
In a study that used a rate-intensity ICSS procedure, 2 and 4 mg/kg of amphetamine shifted the curve to the left, indicating enhanced rewarding efficacy of brain stimulation (96). Consistent findings were observed in the autotitration procedure, in which D-amphetamine (0.03–1 mg/kg, IP) dose-dependently lowered thresholds (138). In a variant train duration procedure, amphetamine, administered at three different doses (0.33, 0.66, and 1 mg/kg, IP) counterbalanced over 3 consecutive days, dose-dependently lowered ICSS thresholds, indicating a reward-enhancing effect (145).
Using the discrete-trial current-intensity procedure, Markou and colleagues showed that exposure of rats to chronic mild stress for a period of 19 days potentiated the enhancement of lateral hypothalamic brain stimulation reward induced by acute administration of amphetamine (1–6 mg/kg, IP, for 2 days) compared with nonstressed animals (143). In a recent study using the rate-frequency curve-shift procedure, acute administration of D-amphetamine into the NAcc shell (1 μg/side) significantly lowered thresholds measured by decreases in M 50 values and did not affect maximal rates of responding (141).
Withdrawal from D-amphetamine decreases brain stimulation reward. Amphetamine administered three times per day for 5 days had an anhedonic effect, indicated by decreases in response rates of electrical brain stimulation when rats were tested 24 h following discontinuation of amphetamine administration and for at least 5 days (171). In studies by Markou and colleagues using the discrete-trial current-intensity procedure, continuous amphetamine exposure using 6-day subcutaneous osmotic minipumps (5, 10, and 15 mg/kg/day) dose-dependently lowered brain reward thresholds and decreased response latencies during two consecutive amphetamine exposures (163). By contrast, cessation of administration of the same amphetamine doses delivered via minipumps for 6 days dose-dependently elevated thresholds, an effect that lasted for 5 days for the highest amphetamine dose (163). Termination of intraperitoneal administration of a variety of amphetamine doses (1–5 mg/kg, three times daily for 1, 2, 4, or 6 days) led to ICSS threshold elevations (173). In a study by Markou and colleagues (164), amphetamine was administered intraperitoneally three times per day for 4 days in a rising-dose regimen starting from 1 mg/kg and stabilizing at 5 mg/kg. Thresholds and response latencies were determined for up to 156 h after the last administration in the discrete-trial current-intensity threshold ICSS procedure. Amphetamine withdrawal resulted in decreased reward, reflected in elevated brain reward thresholds, and these effects were alleviated by co-administration of the selective serotonin reuptake inhibitors fluoxetine or paroxetine and the serotonin 5-HT1A receptor antagonist p-MPPI, indicating that decreased serotonergic function may mediate the effects of amphetamine withdrawal (164, 167). Using a rate-intensity ICSS procedure and stabilizing amphetamine doses at 10 mg/kg (1–10 mg/kg, IP, three times per day for 4 days), Barr and colleagues found that rats exhibited decreased levels of ICSS responding for up to 60 h after the last amphetamine administration, an effect that was counteracted by repeated administration of electroconvulsive shock (172). Previous studies have also reported similar effects of amphetamine withdrawal after cessation of repeated intraperitoneal administration of amphetamine (162, 168, 170, 173).
< div class='tao-gold-member'>
Only gold members can continue reading. Log In or Register a > to continue
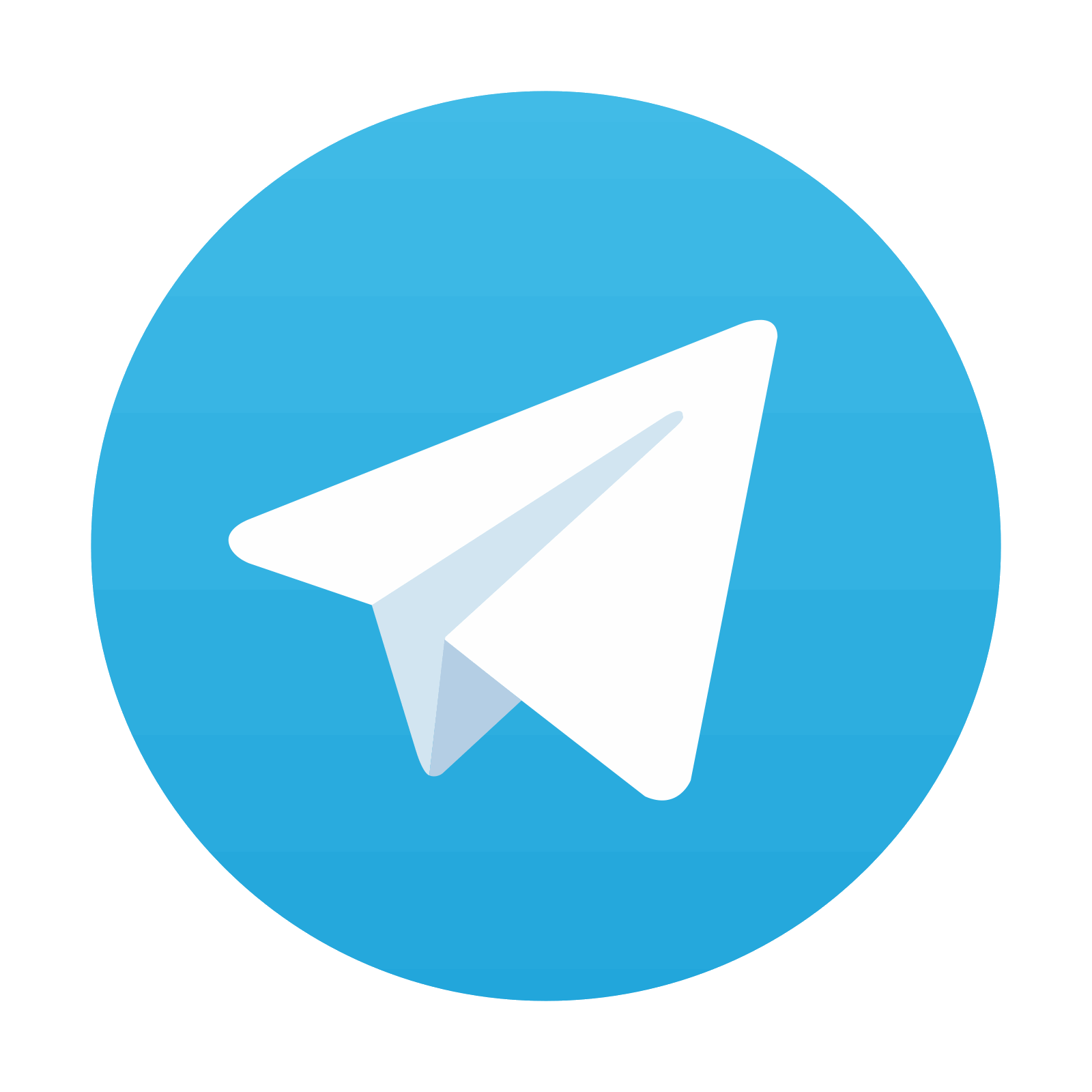
Stay updated, free articles. Join our Telegram channel
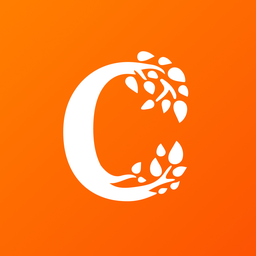
Full access? Get Clinical Tree
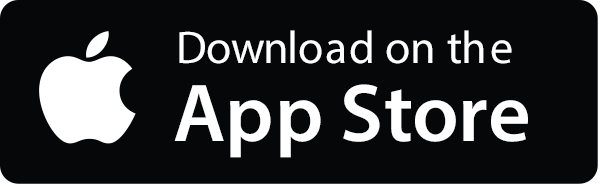
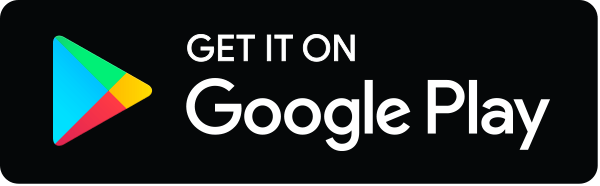