12 Lysa P. Posner Injectable anesthetics provide a rapid means of producing sedation or anesthesia in veterinary patients. The four stages of anesthesia used to define central nervous system (CNS) depression are similar in a patient regardless of whether the anesthetic is injected or administered by inhalation. An advantage of injectable anesthetics, particularly when administered intravenously (IV), is the ability to proceed more rapidly through or bypass stage II anesthesia (the excitement stage). This allows for a rapid and smooth induction of anesthesia, which is aesthetically more pleasant for small animals and safer for personnel in large animals. There is no anesthetic agent that produces ideal anesthesia under all circumstances. When evaluating an injectable anesthetic agent, it is important to evaluate both the pharmacokinetics (absorption, distribution, metabolism, and excretion) and the pharmacodynamics (the behavioral and physiological effects) of a particular drug in order to select an agent most suited to a particular clinical condition, in a particular species. Intravenous anesthetics produce rapid unconsciousness which is advantageous for the following reasons. Rapid induction of anesthesia allows for prompt intubation and control of the airway. Intubation can prevent aspiration, facilitates assisted ventilation, and the administration of oxygen, with or without an inhalant anesthetic. Injectable anesthetics allow for unobstructed visualization of the upper airway for examination or surgical procedures and well as endoscopic access to the upper and lower airways. Some IV anesthetics are anticonvulsant and can provide rapid control and reduction in CNS activity in seizing patients. Induction of anesthesia with injectable anesthetics is less stressful for most patients compared with an inhalant induction and attenuates the excitement seen during stage II anesthesia. For large animals (e.g., horses), preventing the excitement stage is paramount for patient and medical personnel safety. Total intravenous anesthesia (TIVA) techniques are increasing in popularity because they can decrease the stress response, are associated with better recoveries, provide cardiovascular stability, and maintain cerebral autoregulation better than inhalants, without the need for expensive anesthetic equipment. This is a particularly attractive method when anesthetizing animals in the field. Intramuscular (IM) injection of anesthetics can be advantageous for fractious animals or for wildlife or exotic animals, where injectable agents can be administered remotely (e.g., dart or syringe pole). Finally, injectable anesthesia decreases the exposure of people and the environment to inhalant gases. In general, injectable anesthetic agents have a large volume of distribution and wake-up is associated with redistribution and then metabolism of the drug. Thus, it can be hard to fine-tune anesthetic depth and wake-up compared with the inhalant drugs that are generally excreted unchanged through the lungs quite rapidly. However, some of the currently available intravenous agents (e.g., propofol) have such short durations of action that depth of anesthesia can be readily adjusted by changing the administration rate. These types of drugs have made it possible for safe and satisfactory anesthesia without the use of inhalation agents. Another potential disadvantage of injectable anesthetics is the potential for human abuse. Therefore, many injectable anesthetic agents, as well as agents commonly used concurrently with them, are classified as controlled substances under the 1970 US Controlled Substances Act. This places restrictions on purchase, storage, record keeping, and use of these drugs. (Proper handling of Controlled Substances is discussed in Chapter 56 of this book). There is no ideal injectable (or inhalation) anesthetic drug available at this time. One can, however, hypothesize what properties such a drug would have. These can be divided into physiological and pharmacological properties. The ideal injectable anesthetic agent should produce unconsciousness and amnesia, as well as provide analgesia and muscle relaxation. The ideal drug would produce those qualities while maintaining physiological homeostasis; that is, there should be no adverse changes in cardiovascular, respiratory, gastrointestinal, CNS, or endocrine functions. An ideal injectable anesthetic should have a wide margin of safety (high therapeutic index) in a variety of species. It should have a short duration of action and be noncumulative. The drug should be readily excreted unchanged, ideally by more than one route. A specific and complete reversal agent should be available. The ideal drug would be chemically stable, have a long shelf life, have a physiological pH, have a nontoxic vehicle, and would be inexpensive. Barbituric acid was first synthesized in 1864 by Adolph von Baeyer, but the compound was considered clinically useless (Short, 1983). In the early 1900s, the barbiturates or drugs derived from barbituric acid were shown to produce sleep and prevent seizures, and an anesthetic revolution took place. Barbiturates are CNS depressants and can be used at increasing doses to produce sedation, hypnosis, anesthesia, coma, and even death (euthanasia solutions). The major uses for barbiturates in veterinary medicine are as induction agents for anesthesia, anticonvulsants, and euthanasia agents. Although short-acting barbiturates are still commonly used for anesthesia in both veterinary and human medicine worldwide, they are no longer available in the USA. The European distributors of barbiturate anesthetics will no longer sell them to the USA due to objections regarding their use in human executions (NPR, 2014). Barbiturates for use as anticonvulsants and for veterinary euthanasia solutions are still available in the USA. Euthanasia solutions are discussed in Chapter 16. Barbiturates are derivatives of barbituric acid, which is a combination of malonic acid and urea (Figure 12.1, barbituric acid). The addition of an alkyl or aryl group at position 5 of the barbituric acid ring imparts CNS depressant effects. Further modification of the molecule by substituting sulfur (thio) for oxygen (oxy), at position 2 of the barbiturate acid ring, increases the lipid solubility (thiobarbiturates vs. oxybarbiturates) (Figure 12.1, thiopental and methohexital). Lipid solubility can also be enhanced by increasing the number of side chains at position 5a and 5b of the barbituric acid ring (Figure 12.1, thiopental). Increasing lipid solubility results in a decreased duration of action (shorter acting), an increased metabolic degradation, and an increased hypnotic potency (Figure 12.1, methohexital). Longer side chains at position 5 increases anticonvulsant activity (Figure 12.1, phenobarbital, pentobarbital). Figure 12.1 Barbiturates and derivatives of barbituric acid. Barbiturates are often classified by either their duration of action (e.g., long, intermediate, short, or ultrashort) or by their chemical structure. The chemical structure (e.g., oxybarbiturates, thiobarbiturates) of barbiturates determine the time of onset and duration of action, which in turn determines their use (Table 12.1). Table 12.1 Classification of barbiturates by type of barbiturate and common use Barbiturates are poorly soluble in water at physiological pH. However, through chemical manipulation, they can be made to form water-soluble salts when added to alkaline aqueous solutions. These salts hydrolyze in water to form highly alkaline solutions with a pH usually between 9 and 10. If the high pH is not maintained (for example if mixed with acidic solutions) precipitation can occur. Thus although barbiturates are chemically acids they are prepared as salts that are bases. The high alkalinity of the solution makes it caustic if given perivascularly (can cause sloughing of tissue) but makes the solution fairly bacteriostatic. Barbiturates are delivered as a racemic mixture with their L-isomers nearly twice as potent as their D-isomers. Barbiturates are γ-aminobutyric acid (GABAA) receptor agonists which enhance inhibitory neurotransmission. They increase GABA binding (primary inhibitory neurotransmitter) by decreasing the rate of dissociation of GABA from its receptor. Activation of the GABA receptors increases transmembrane chloride conductance, resulting in hyperpolarization of the postsynaptic cell membrane, which causes inhibition of the postsynaptic neuron. Barbiturates also block glutamate binding (main excitatory neurotransmitter) at AMPA receptors. Barbiturates depress the reticular activating system (RAS), which controls arousal and inhibit the development or spread of epileptiform activity. The RAS system is particularly sensitive to barbiturates (Harvey, 1975) and animals are unable to be aroused or to maintain the wakeful state following administration. Furthermore, at high doses, barbiturates can decrease transmission of nerve impulses at nicotinic acetylcholine receptors in the neuromuscular junction (Arias et al., 2006). These effects occur because barbiturates decrease the sensitivity of polysynaptic junctions to the depolarizing action of acetylcholine, which results in muscle weakness. Barbiturates are used in veterinary medicine for rapid induction of general anesthesia, as anticonvulsants, and for euthanasia (for use as anticonvulsants and euthanasia solutions see Chapters 16 and 17). Thiopental is the most commonly used barbiturate in veterinary medicine worldwide. It is an ultrashort-acting thiobarbiturate and differs from pentobarbital only at the number 2 carbon where a sulfur molecule has replaced the oxygen (Figure 12.1). The drug is prepared as a sterile powder and after reconstitution with an appropriate diluent is administered by the intravenous route. Thiopental sodium is chemically designated sodium 5-ethyl-5-(1-methylbutyl)-2-thiobarbiturate. The drug is a yellowish, hygroscopic powder, stabilized with anhydrous sodium carbonate as a buffer (60 mg/g of thiopental sodium). Thiopental is dispensed as a powder buffered with sodium carbonate which is commonly reconstituted with sterile water or sterile saline. Once reconstituted, thiopental has a pH between 9 and 10, which is caustic if administered perivascularly. The high alkalinity of the solution results in thiopental having a long shelf life, but as the solution ages it becomes less potent (Thurmon et al., 1996). Although supplied in an alkaline pH, thiopental is a weak organic acid with a pKa value of 7.6, and has a relatively low ionization (39%) at plasma pH (Brandon and Baggot, 1981). The nonionizing moiety is highly lipid soluble, which allows for it to quickly penetrate the blood–brain barrier and thus it is a rapidly acting anesthetic. In general, the 2.5% solution is used with small animals and the 2.5% or 5% solution is used with larger animals. Thiopental can be mixed with propofol in a 1 : 1 (vol : vol) ratio, which is chemically stable (Paw et al., 1998). The combination of the two GABA agonists produces a synergistic clinical effect and improves the induction and recovery profile of thiopental to more resemble that of propofol (Ko et al., 1999). Thiopental, like most barbiturates, undergoes extensive liver metabolism. Thiamylal was an ultrashort-acting thiobarbiturate commonly used in veterinary medicine, but is no longer commercially available. Information on this drug can be found in earlier editions of this book. Pentobarbital is a short-acting oxybarbiturate that has been used in veterinary medicine since the early 1930s. Although commonly used in the past for clinical anesthesia, it current use is primarily as a euthanasia solution (see below in this section) and for anesthesia in laboratory rodents. Pentobarbital is effective when given intraperitoneally as well as intravenously and thus can be used successfully in small rodents. It undergoes extensive hepatic metabolism and is totally dependent on the liver for biotransformation and elimination. Animals can show excitement during induction and recovery, which can be prevented by concurrent use of tranquilizers or sedatives. The duration of action of pentobarbital is much longer (four to eight times) than thiopental in most animals and therefore is therefore less commonly used for anesthesia. However, its long duration of action makes it clinically useful for anticonvulsant treatment. Pentobarbital is the primary ingredient in most commercially available euthanasia solutions. See Chapter 16 for more detailed discussion of euthanasia solutions. Lidocaine and phenytoin are often added to increase cardiovascular depression as well as change the solution from a schedule II controlled drug to a schedule III controlled drug. The lethal dose of pentobarbital in the dog is 85 mg/kg orally and 40–60 mg/kg intravenously. Toxicosis, including death, has been reported in dogs fed uncooked meat from a horse euthanatized 8 days previously with pentobarbital (Polley and Weaver, 1977). Cooking does not inactivate pentobarbital in meat, as animals euthanatized with pentobarbital and rendered at a commercial plant showed virtually no degradation of the drug (O’Connor et al., 1985). The recommended dose for euthanasia is 87 mg/kg IV. Phenobarbital was first synthesized in Germany in 1912 and was marketed as Luminal. It is a long-acting oxybarbiturate, whose current primary use is as an anticonvulsant or for long-term sedation (e.g., for treatment of animals with strychnine poisoning or tetanus). More information about the anticonvulsant use of phenobarbital is in Chapter 17. Methohexital is a unique oxybarbiturate in that it is ultrashort acting. Methohexital is twice as potent as thiopental (Turner and Ilkiw, 1990) but has a higher incidence of CNS excitatory effects. Methohexital activates abnormal EEG tracings in epilepsy-prone subjects (Musella et al., 1971). Methohexital should not be used in patients with increased CNS excitation (e.g., strychnine poisoning) or with seizure disorders. Methohexital should be administered intravenously; however, perivascular injection does not produce tissue irritation. Administration of methohexital should be fairly rapid since slow injection rates can be associated with muscular tremors and excitement. Excitement and muscle tremors can be reduced by prior administration of sedatives or tranquilizers. The duration of action of methohexital is short, with dogs and cats attaining sternal recumbency within 5–10 minutes of administration (Sams et al., 1985). Although methohexital is metabolized four to five times faster than thiopental, awakening is due to redistribution rather than metabolism. In Greyhound dogs, recovery from methohexital anesthesia is significantly faster than from thiopental (Sams et al., 1985) and until the introduction of propofol to veterinary practice, methohexital was considered the induction agent of choice for Greyhound dogs. Barbiturates are administered to produce anesthesia (short-acting barbiturates) or as anticonvulsants (long-acting barbiturates). However, they possess properties that are favorable to cerebral physiology. Barbiturates decrease cerebral blood flow (CBF) (Albrecht et al., 1977) and cause a dose-dependent depression of the electroencephalogram (EEG), eventually resulting in a flat EEG (Kiersey et al., 1951). They decrease cerebral oxygen consumption (CMRO2) particularly in cortical areas by up to 55%, which parallels the depression of the neuronal activity. Metabolic needs of the brain remain constant; only hypothermia will decrease cerebral metabolic needs (Steen et al., 1983). Furthermore, they decrease intracranial pressure (Bedford et al., 1980) and similarly decrease intraocular pressure (Mirakhur and Shepherd, 1985). Since intracranial pressure (ICP) decreases more than mean arterial pressure (MAP), cerebral perfusion pressure is preserved. Thus barbiturates like thiopental are often chosen to anesthetize patients with CNS disease or trauma. Methohexital, is an ultrashort-acting barbiturate, but is an exception to the cerebro-friendly status of barbiturates. Methohexital has been associated with generalized excitement and activation of epileptic foci (Stoelting, 1999) and is not recommended for patients with a seizure history. Barbiturates cause changes in the cardiovascular system that is dependent on the dose and the physiological status of the patients. In healthy dogs, there is a decrease in stroke volume and contractility with an increase in heart rate (Turner and Ilkiw, 1990). In the healthy dogs, there is a minimal change to cardiac output as the reflex tachycardia compensates for the decrease in stroke volume, but mild hypotension is often seen. Although unproved, anecdotal reports suggest that in compromised dogs, barbiturates result in an exaggerated cardiovascular depression. However, healthy dogs that suffered from acute blood loss did not show a depression in cardiovascular parameters (Ilkiw et al., 1991a). Dogs and pigs do, however, need a smaller induction dose following severe hemorrhage (Weiskopf and Bogetz, 1985). Caution should be used when administering barbiturates to patients with dehydration, hypovolemia, anemia, blood loss, or underlying cardiac disease. Thiopental can precipitate ventricular arrhythmias, particularly bigeminy (Muir, 1977), as well as potentiate epinephrine-induced arrhythmias (Atlee and Malkinson, 1982). Lidocaine can be administered with thiopental. This allows for less thiopental to be administered (reduces cardiovascular depression) and protects against ventricular arrhythmias (Rawlings and Kolata, 1983). Caution should be used when administering thiopental to patients with high sympathetic tone or who are prone to arrhythmias. There is a dose-dependent effect of the vasomotor center by barbiturates. Rapid IV injection of an induction dose of a thiobarbiturate causes a sharp but transitory fall in arterial pressure because of the high concentration briefly depressing the vasomotor center. Venodilation results in splenic sequestration of red blood cells (RBC) and a concomitant drop in packed cell volume. For this reason barbiturates are often avoided for anesthesia of patients scheduled for a splenectomy (Baldo et al., 2012). Furthermore, the vasodilation contributes to heat loss. With a decline in heat production during anesthesia and an increase in heat loss owing to peripheral vasodilation, anesthetized patients can quickly become hypothermic. Barbiturates do not block the autonomic responses to pain or intubation and thus should be administered with drugs that will attenuate those responses (e.g., opioids). When used at anesthetic induction doses, barbiturates produce central respiratory depression. There is a decrease in respiratory rate and minute ventilation, with apnea in some patients (Quandt et al., 1998). In addition to causing respiratory depression, the barbiturates decrease the reflex responses to hypercapnia and hypoxemia (Hirshman et al., 1975). Following induction with a barbiturate, patients should be intubated and assisted with ventilation as necessary. The blood concentration inhibiting the respiratory center is considerably less than that arresting the heart, so patients that are apneic may be stable cardiovascularly. The occurrence of bigeminy appears to be decreased in well-oxygenated patients. Oral barbiturates used as anticonvulsants have minimal or no effect on respiratory function, probably due to lower plasma concentrations. Barbiturates produce good muscle relaxation and generally produce favorable intubating conditions. Laryngeal reflexes are preserved with barbiturate anesthesia (Jackson et al., 2004) facilitating better evaluation of laryngeal function. The increased sensitivity of laryngeal and bronchial reflexes can lead to laryngospasm (Barker et al., 1992). Although laryngeal reflexes are preserved, all patients induced with a barbiturate should be intubated to protect the airway and support ventilation. There are no important effects such as diarrhea or intestinal stasis following routine use of thiobarbiturates. The thiobarbiturates have no direct effect upon the kidney, but can decrease urine output because of decreased blood flow and glomerular filtration rate resulting from hypotension and decreased cardiac output. Patients with uremia have decreased plasma binding of barbiturates (more physiologically available drug) and thus need lower induction doses (Ghoneim and Pandya, 1975). Most barbiturates easily cross the placenta and equilibrium between maternal and fetal circulations is established within a few minutes. While in utero, most fetuses are slightly acidemic when compared to the mother. Since barbiturates are less ionized in an acid environment, it is possible that the neonate will have a greater concentration of active drug. In humans, anesthesia with thiopental for cesarean sections resulted in neonates with depressed reflexes, altered acid–base status, and delayed suckling when compared to epidural anesthesia (Sener et al., 2003). Similarly, neurological function was depressed in puppies following cesarean sections where the dam was administered thiopental (Luna et al., 2004). Pentobarbital has been associated with slow neonatal recovery, delayed nursing, and increased morbidity (Thurmon et al., 1996). Phenobarbital is excreted in the milk, although the amounts are marginal. However, a suckling neonate can ingest the drug passed from the mother (Wong et al., 2008). Because neonates may have impaired clearance compared to an adult, this can potentially lead to adverse effects (sedation and decreased suckling activity). Doses of thiobarbiturates that produce surgical anesthesia depress basal metabolism so that less body heat is produced during anesthesia concurrently with excessive heat loss as a result of vasodilation. It is important that surgical patients anesthetized with barbiturates be kept warm. The decrease in vasomotion associated with barbiturates also leads to splenic sequestration of RBCs. Since the spleen can become quite large and a significant amount of blood and be stored in the spleen, barbiturates are not recommended for patients having a splenectomy. The “glucose effect” is a term used to describe a reanesthetizing action seen in animals recovering from barbiturate anesthesia that are administered glucose solutions. Glucose decreases microsomal metabolism and thus can decrease barbiturate metabolism (Peters and Strother, 1972). A similar event can occur with administration of epinephrine; however, little clinical effect is seen at routine barbiturate doses (Hatch, 1966). Barbiturates do not provide analgesia. While anesthetized, nociceptive input is not perceived by the brain, but the pain pathways are activated. In patients where pain will continue following anesthesia, alternative analgesics must be used. At subanesthetic doses, barbiturates may cause hyperalgesia (Ewen et al., 1995), but this effect is controversial and probably not clinically relevant when barbiturates are used as induction agents. Thiopental is highly protein bound (>70%) (Brandon and Baggot, 1981) and has a pKa near 7.6. After IV administration, thiobarbiturates are initially present in high concentrations in highly perfused tissues (e.g., the brain), resulting in rapid induction of general anesthesia. Thiobarbiturates then redistribute to the moderately perfused body tissues (e.g., muscle). This redistribution to moderately perfused tissues decreases the concentration in the plasma in brain tissues allowing the animal to regain consciousness. Further redistribution to adipose tissue from both highly and moderately perfused tissues results in the complete recovery from thiobarbiturate anesthesia. Animals with lower percentages of fat and/ or muscle tissue have a smaller percentage of area for the barbiturates to redistribute to. Thus normally lean patients (e.g., Sighthounds), neonates, or cachetic patients might have prolonged recoveries from thiobarbiturates. Similarly, since awakening depends of redistribution, repeated dosing is not recommended because of cumulative effects and prolongation of recovery. Pharmacokinetics of thiopental in rabbits, sheep, and dogs showed an central volume of distribution of 38.6 ± 10 ml/kg, 44.5 ± 9.1 ml/kg, and 38.1 ± 18.4 ml/kg, respectively (Ilkiw et al., 1991b). Elimination half-life was longest in the sheep (251.9 ± 107.8 min), shorter in the dog (182.4 ± 57.9 min), and shortest in the rabbit (43.1 ± 3.4 min) (Ilkiw et al., 1991b). The activity of a drug is dependent on the percentage of the drug that is unbound and unionized. Since thiopental is an acid it is nonionized in an acid environment. It is also highly protein bound. Many ill patients are both acidemic and hypoproteinemic and thus may have greater percentage of active drug available for pharmacological activity. Thus, the therapeutic index may be smaller in ill patients, making them easier to overdose. Phenobarbital is readily absorbed from the GI tract with bioavailability ∼90% in dogs (Pedersoli et al., 1987) and ∼99% in horses (Ravis et al., 1987). Pharmacokinetics of an IV bolus dose of phenobarbital in horses showed a volume of distribution at steady state of 0.803 ± 0.07 l/kg; terminal phase elimination of 18.3 ± 3.65 hours; and total body clearance 30.8 ± 6.2 ml/kg/h (Duran et al., 1987). In the dog, volume of distribution is ∼0.7 L/kg; the mean half-life was 92.6 ± 23.7 hours; mean total clearance 5.6 ± 2.31 ml/h/kg (Pedersoli et al., 1987). In the goat, the volume of distribution for pentobarbital (30 mg/kg) administered intravenously is 0.72 l/kg; the first-order disappearance rate kinetic constant is 0.76 hour and the half-life is 0.91 hour (Boulos et al., 1972). In dogs, the elimination phase half-life of pentobarbital is 8.2 ± 2.2 hours. The steady-state volume of distribution is 1.08 ± 0.21 liters/kg and the elimination clearance is 0.0013 ± 0.0004 liters/min (Frederiksen et al., 1983). It is 35–45% protein bound. Comparatively, ruminants, especially sheep and goats, metabolized pentobarbital much faster; elimination half-life in goats is ∼1 hour compared to ∼8.0 hours in dogs. Barbiturates are almost completely biotransformed in the liver and eliminated by the kidney. Most barbiturates are metabolized principally by the hepatic microsomal enzyme system and cause microsomal enzyme induction (CYP450 system). This can result in altered metabolism of other drugs as well of the barbiturate itself if given repeatedly. Neonates, cachetic, or hypothermic animals can have decreased microsomal metabolism and thus these drugs may have a prolonged effect in these animals. Recovery times for patients with hepatic dysfunction can be markedly prolonged, but fairly normal in patients with renal dysfunction. Although awakening from thiobarbiturates is generally more rapid than from oxybarbiturates (except for methohexital), this is due to rapid redistribution rather than from rapid metabolism. Greyhounds are deficient in oxidative enzymes needed for metabolism of thiobarbiturates (Robertson et al., 1992). Thus along with having low fat stores, metabolism is extremely prolonged and awakening may be >8 hours. Thiobarbiturates should not be used in Greyhounds. Tolerance has been reported with multiple or frequent use of barbiturates. It can occur as a sequela to the induction of microsomal enzyme activity (i.e., increased metabolism) or as a general neurological adaptation to chronic barbiturate effects. Barbiturates can cause sequestration of RBC in the spleen from venodilation. Thus, these drugs are often avoided for a splenectomy. Thiobarbiturates should not be used in Greyhound dogs (see Section Metabolism). Perivascular administration of thiobarbiturates can result in tissue damage and necrosis (sloughing). Infiltration with saline and lidocaine has been recommended for treatment of perivascular injections (Tuohy and MacEvilly, 1982). Table 12.2 shows dose ranges to produce anesthesia for common barbiturates in different species. Lower doses are generally used when patients are premedicated with other CNS depressant or have serious concurrent disease and higher doses are generally needed when no other drugs are given. The use of premedicants with barbiturate anesthesia produces a smoother induction and recovery. Table 12.2 Doses of common barbiturates in various species a Dose of pentobarbital for euthanasia is 87 mg/kg. IP, intraperitoneal. The pH of thiopental is ∼10 and therefore should only be given IV, as any injection outside of the vein can lead to pain, tissue injury, and skin sloughing. Intraarterially, barbiturates, in particular thiopental, produce spasm of the arterial wall, which can lead to thrombosis and poor perfusion if collateral circulation is not present (e.g., pinnae of ear, digits). Thiopental should not be administered into body cavities. Thiopental should be administered “to effect.” That is, the entire dose is calculated but given slowly until the desired effect is present; usually the end-point is a patient that can be easily intubated. The slower-acting barbiturates need to have a portion of the dose “bolused” to prevent seeing the excitement stage. If thiopental is given after premedication, this quick bolus is unnecessary. Most barbiturates have a similar but small therapeutic index (lethal dose/ therapeutic dose); however, the shorter-acting barbiturates have fewer postanesthetic complications because of their shorter duration of action. Since World War II, warnings have been issued about the increased mortality with the use of barbiturates in trauma patients (Halford, 1943). Trauma or critically ill patients are more likely to be acidemic and hypoproteinemic and thus will have an increased fraction of unionized (active) drug available. Uremia can also decrease protein binding and increase the percentage of active drugs. Dogs and pigs with acute blood loss need smaller induction doses (Weiskopf and Bogetz, 1985). Thus these patients are more easily overdosed and care should be used to assure appropriate dosing. However, with proper dose adjustments, the risks for barbiturate use with critically ill patients can be minimized and these drugs can be used safely (Bennetts, 1995). Duration of action of thiopental in dogs is fairly short with extubation occurring 25.2 ± 15.7 min after an induction dose and ability to walk unaided at 72.8 ± 26.1 minutes (Quandt et al., 1998). Since all of the barbiturates require extensive liver metabolism, it should be expected that neonates and animals with hepatic dysfunction would have a prolonged duration of action. There is also significant individual variation based on sex, weight, nutritional status, body temperature, and breed as well as the type of barbiturate used. For example, thiobarbiturates have a longer duration in Greyhound dogs than in mixed breeds (Robertson et al., 1992). Alternatively, methohexital, an oxybarbiturate, induces a shorter period of anesthesia in the Greyhound dogs than thiopental, a thiobarbiturate (Robertson et al., 1992). Various reasons have been given for the differences in Greyhound dogs as compared to other breeds (Robinson et al., 1986; Court, 1999). Chloramphenicol can double the duration of action of pentobarbital because it inhibits the microsomal enzymes responsible for metabolism of some barbiturates. Since awakening from thiobarbiturates is primarily due to redistribution rather than metabolism, the concurrent administration of chloramphenicol with thiopental does not prolong awakening when both drugs are used at clinically relevant doses (Reich et al., 2005). Since awakening from anesthesia is due to redistribution of barbiturates to fat and muscle, lean breeds with small fat stores (e.g., Whippet, Irish Wolfhounds) can show a prolonged effect to barbiturates. With little area to redistribute the drug, these breeds also have an increased risk of overdose. Greyhounds are classified as Sighthounds and are at risks as discussed above. In addition, Greyhounds are deficient in oxidative enzymes needed for metabolism of thiobarbiturates and thus can have extremely prolonged recoveries (Robinson et al., 1986). Although not toxic, the increased risk of overdose coupled with the prolonged recovery makes thiopental not recommended for use Greyhounds. In Greyhounds, recovery from methohexital anesthesia is significantly faster than from thiopental (Sams et al., 1985). Currently, propofol is more commonly used in Greyhounds for anesthesia than barbiturates. Barbiturates can be successfully used for anesthesia in ruminants, but because of the propensity for ruminants to regurgitate, the airway needs to be protected by placement of a cuffed orotracheal tube. In cattle, a congenital condition (“pink tooth”) that increases porphyrin concentrations can occur, which can lead to neurological disturbances from demyelination of peripheral and cranial nerves. In the liver, barbiturates stimulate production of an enzyme that increases porphyrin production potentially making the disease worse (Mees and Frederickson, 1975). Animals afflicted with a known or suspected disturbance in porphyrin metabolism should not be administered barbiturates. Many pigs may have prolonged recoveries from thiopental administration (∼1 hour) versus other animals (∼15 minutes). It is possible that redistribution of thiopental to the increased fat stores may contribute to the prolonged effect of the drug. Barbiturates do not trigger malignant hyperthermia. The administration of thiopental to horses without prior sedation is not recommended as there is significant excitement and incoordination. Rather, horses should be administered thiopental following sedation with an α2-agonist (e.g., xylazine) and/or guaifenesin, and/or a benzodiazepine (e.g., diazepam). Induction of anesthesia with thiopental causes horses to become recumbent by the flexion of all four legs, as compared to ketamine where most horses will flex their hind legs first and assume a dog-sitting posture before complete recumbency. Care should be taken to prevent horses from injuring themselves during induction and recovery. Methohexital should not be used alone in horse because excitation during induction and/ or recovery. Thiopental has been successfully used in birds interosseously (Valverde et al., 1993), but because of prolonged recoveries and increased mortality it is not recommended. Barbiturates are metabolized very slowly in reptiles resulting in a prolonged recovery. The ultrashort-acting barbiturate, methohexital, has been used with varying duration of action in snakes (Preston et al., 2010). All CNS depressants (e.g., opioids, α2-agonists, phenothiazine sedatives) can potentiate the effects of barbiturates, reducing the required induction dose and increasing the risks of physiological side effects. Long-acting barbiturates induce hepatic microsomal enzymes, which increases the metabolism of other drugs metabolized by the same system (e.g., chloramphenicol, beta-blockers, and metronidazole). The induction of microsomal enzymes does not occur after a single dose. Therefore, the likelihood of this occurrence is low with anesthetic uses. However, it is common when repeated oral doses are administered such as when phenobarbital is administered as an anticonvulsant in animals. The other important interaction that can occur is through microsomal enzyme inhibition. (Anticonvulsant drugs are covered in more detail in Chapter 17.) As discussed in Section Metabolism, drugs known to be CYP450 inhibitors, such as chloramphenicol, can inhibit the metabolism of barbiturates and prolong recovery. Treatment for an overdose with short-acting barbiturates should center on supportive treatment of respiratory and cardiovascular depression. This may include positive pressure ventilation, fluid therapy, and inotropic support. Clearance of long-acting barbiturates is considerably greater in alkaline than in acid urine. This occurs because alkaline urine produces ionization of the drug and prevents tubular reabsorption. Thus alkalinization of urine with sodium bicarbonate increases the elimination rate and is clinically useful in treatment of intoxication from long-acting barbiturates. If a known perivascular injection occurs, the area should be infiltrated with saline for dilution and acidification and a local anesthetic to decrease pain. Propofol is an intravenous anesthetic used for sedation, induction of anesthesia, or maintenance of anesthesia when administered as a constant rate infusion (CRI). It has been approved for human use since 1989 and is popular in veterinary medicine due to its smooth induction and recovery. It is unusual in that it is supplied in a milky white emulsion that is administered intravenously. Propofol (2,6-diisopropylphenol) (Figure 12.2) is an alkyl phenol derivative that is water insoluble but highly lipid soluble. Propofol is chemically described as 2,6-diisopropylphenol and has a molecular weight of 178.27. Propofol is very slightly soluble in water and is therefore formulated as a white, oil-in-water emulsion. Figure 12.2 Structure of propofol. There are a number different propofol formulations marketed to the human and veterinary markets. Most of the formulations are similar; however, some contain small amounts of EDTA or benzyl alcohol. PropoFlo™ Injection is a sterile, nonpyrogenic emulsion containing 10 mg/ml of propofol suitable for intravenous administration. In addition to the active component, propofol, the formulation also contains soybean oil (100 mg/ml), glycerol (22.5 mg/ml), egg lecithin (12 mg/ml), and oleic acid (0.6 mg/ml) with sodium hydroxide to adjust the pH. The propofol emulsion is isotonic and has a pH of 6.0–9.0. The formulation available contains no preservatives and will support bacterial growth (Wachowski, 1999). Since propofol is an emulsion it should be shaken well before using. Propofol with extended shelf life, PropoFlo™ 28, is a sterile, nonpyrogenic emulsion containing 10 mg/ml of propofol in a multidose vial for intravenous administration. The name “PropoFlo 28” is used because, compared to previous formulations, it has a 28-day shelf-life once the vial is opened. This is designed to reduce anesthetic waste and no refrigeration is required for the 20-ml and 50-ml vial sizes. Each ml contains 10 mg propofol. The PropoFlo™ 28 emulsion is isotonic and has a pH of 6.0–8.5. PropoFlo 28 is approved for use in dogs, only. The effects of the benzyl alcohol preservative in cats have not been studied and therefore cannot be recommended. Propoclear® was a propofol formulation that did not use a lipid carrier. The solution was clear and pharmacodynamic effects were similar to standard formulations of propofol. However, the product was associated with increased injection site reactions and was removed from the market (Hill and Williams, 2011). Propofol produces CNS depression by its effect on the GABAA receptor (Ying and Goldstein, 2005). Propofol decreases the rate of GABA dissociation from its receptors thus increasing the opening of chloride channels. Increased calcium conductance results in hyperpolarization of postsynaptic cell membranes and inhibition of the postsynaptic neurons resulting in anesthesia and amnesia. Although shown to be anticonvulsant in veterinary and human patients with variable CNS disease (Brown and Levin, 1998; Steffen and Grasmueck, 2000), the mechanism of action as an anticonvulsant is unknown. Clinical uses of propofol include: induction of general anesthesia, short-term sedation, long-term sedation (e.g., ventilator patients), maintenance of anesthesia (via constant rate infusion), and treatment of status epilepticus. Propofol should only be administered intravenously; however, extravasation does not cause skin sloughing as it does with barbiturate anesthetics. Propofol induces CNS depression by enhancing the effects of GABA, an inhibitory neurotransmitter, providing a rapid, smooth induction of general anesthesia. Propofol produces a favorable neurological status as it decreases ICP, decreases cerebral perfusion pressure, and decreases cerebral metabolic oxygen consumption (Pinaud et al., 1990). Since cerebral metabolic demands are decreased more than blood flow decreases, perfusion is adequate. Intracranial pressure is reduced in patients with both normal and elevated ICP. Propofol has also been shown to maintain cerebral autoregulation in pigs (Lagerkranser et al., 1997) and increase cerebral autoregulation in humans (Harrison et al., 2002). Similar to its effects on intracranial pressure, propofol causes an acute decrease in intraocular pressure by 30–40% when administered at anesthetic doses (Neel et al., 1995). Myoclonus has been reported following propofol administration and some have hypothesized that propofol might lower the seizure threshold. However, propofol has been shown to be anticonvulsant and does not produce seizure activity in patients with documented epilepsy (Cheng et al., 1996). Propofol has been used successfully in both dogs and humans for long-term control of status epilepticus (Brown and Levin, 1998). Following propofol anesthesia, many human patients report a feeling of euphoria as well as having had amorous dreams (Canaday, 1993). Many domestic animals recover from propofol clear headed, coordinated, and seemingly feeling well. The combination of good CNS perfusion, good quality recovery in patients with CNS disease (Caines et al., 2014), and anticonvulsant properties, makes propofol is a popular anesthetic for patients with CNS disease. Induction doses of propofol are associated with systemic hypotension (Reich et al., 2005). Although propofol causes negative inotropy, the decrease in blood pressure is primarily via a decrease in systemic vascular resistance (vasodilation); systemic vascular resistance decreased by 21% in dogs following an induction dose of propofol (5 mg/ml) (Wouters et al., 1995). The decrease in system vascular resistance likely contributes to splenic engorgement and this should be considered in patients anesthetized for splenectomy (Baldo et al., 2012). Following an induction dose of propofol, the heart rate is often decreased or unchanged, even with systemic hypotension (Whitwam et al., 2000). This is contrast to the barbiturates where there is often an increased heart rate in response to the decrease in blood pressure. Therefore a decrease in cardiac output should be expected. Propofol can enhance the ability of epinephrine to induce cardiac arrhythmias but does not appear to be inherently arrhythmogenic (Kamibayashi et al., 1991). Apnea is a commonly seen following bolus administration of propofol. The apnea associated with propofol is increased as the rate of infusion is increased. That is, the faster the administration the more likely the incidence of apnea (Musk et al., 2005). Respiratory depression resulting in mild hypercapnia and acidosis are also seen in spontaneously breathing dogs following induction doses (Robertson et al., 1992) and in dogs administered propofol as a constant rate infusion. Patients given propofol should be monitored for hypoxemia and hypercapnia and if necessary the patient should be intubated and provided supplemental oxygen. Propofol should be cautiously in patients where securing an airway may be difficult (e.g., laryngeal masses, guinea pigs, etc.). Propofol provides excellent muscle relaxation, but occasionally results in short and long-term myoclonic movements in humans and dogs (Nimmaanrat, 2005). Signs resolve spontaneously, but severity of movement can interfere with surgical procedures. Anecdotal treatment with ketamine at 1 mg/kg IV has resulted in the resolution of signs, whereas treating with benzodiazepines does not appear to affect the myoclonus (author’s personal experience). Laryngeal reflexes are decreased and result in a favorable intubating environment, but might increase the risk of aspiration in patients without a protected airway. Propofol crosses the placenta and enter the fetal circulation, but it appears to be readily removed from the fetal circulation after birth and produces minimal effects on healthy, newborn, human infants (Dailland et al., 1989) or puppies (Luna et al., 2004). Propofol does not cause histamine release (Mitsuhata and Shimizu, 1993) and results in a diminished stress response compared with inhalant anesthetics (Ledowski et al., 2005). Propofol has antioxidant effects in humans, dogs, and pigs (Aldemir et al., 2001; Allaouchiche et al., 2001; Lee, 2012). The clinical relevance of this activity is unclear. Propofol has been shown to decrease postanesthetic nausea and vomiting in people at both anesthetic and subanesthetic doses (Apfel et al., 2004); however, this effect has not been documented in domestic animals. Subanesthetic IV doses of propofol have been shown to decrease the pruritus seen following spinally administered opioids in humans (Horta et al., 2006). Propofol does not provide any analgesia or produce hyperalgesia as has been reported with barbiturate anesthesia (Wilder-Smith et al., 1995). The pharmacokinetics of propofol in dogs was described using a two-compartment open model (Zoran et al., 1993). Initially, the drug is extensively taken up by the CNS, resulting in rapid CNS depression and induction of anesthesia. It is then rapidly redistributed from the brain to other tissues and removed from the plasma by metabolism. Propofol’s lipophilic nature results in a large apparent volume of distribution (Vd; 17.9 l/kg in mixed-breed dogs) and steady-state volume of distribution (Vdss 9.7 ml/kg). The initial distribution half-life (t1/2α) is short, as is the plasma disappearance (t1/2β), due to rapid redistribution of the drug from the brain to other tissues and extensive metabolism. The Vd and Vdss are smaller in Greyhounds, 11.2 l/kg and 6.3 ml/kg respectively, which will produce higher concentrations at similar doses compared to other breeds, and there may be slower recoveries compared with other dogs (Zoran et al., 1993). Geriatric dogs (>8.5 years) have also been shown to have a slower clearance than that reported for younger dogs (Reid and Nolan, 1996). Slower systemic clearance may also account for the prolonged effects in Greyhounds and geriatric dogs (see Section Metabolism). The pharmacokinetics of propofol in cats has not been fully determined; however, cats appear to have a similar response to and dose requirement as dogs for propofol. Interestingly, it has been shown that in cats there is a significant pulmonary uptake of propofol (Matot et al., 1993). Propofol does not accumulate (noncumulative) after repeated doses and/or prolonged infusions in dogs or laboratory animals (Adam et al., 1980; Mandsager et al., 1995); however, in cats longer constant rate infusions resulted in a longer recovery time (Pascoe et al., 2006). The total body clearance of propofol is rapid and exceeds hepatic blood flow, suggesting extrahepatic metabolism (Veroli et al., 1992). Indeed, in one study performed in human patients undergoing liver transplantation, the amount of propofol metabolite excreted did not decrease when the liver was excluded from the circulation (Veroli et al., 1992) and the pharmacological effects are not different in patients with cirrhosis (Servin et al., 1990). The site of extrahepatic metabolism is unclear, but pulmonary tissue has been shown to contribute to propofol metabolism in cats (Matot et al., 1993). There may be significant species differences in the site and amount of extrahepatic metabolism. Although awakening is generally attributed to redistribution, the termination of the effect of propofol is largely attributed to rapid and extensive biotransformation by the liver to inactive metabolites, which are excreted in the urine. In the dog the two major urinary metabolites of propofol are the glucuronide and sulfate conjugates of 4-hydroxypropofol (2,6-diisopropyl-1,4-quinol) (Hay Kraus et al., 2000). The formation of this intermediate derivative appears to be a critical initial step in the biotransformation and elimination of propofol in dog (Hay Kraus et al., 2000). It has also been shown that there are breed differences in metabolic enzyme pathways, which would account for the slower drug clearance seen in Greyhound and geriatric dogs (Reid and Nolan, 1996; Court et al., 1999; Hay Kraus et al., 2000). Although the amount of hepatic glucuronidation has not been studied in cats, there are reports of delayed recovery in cats after prolonged constant rate infusions of propofol, possibly due to the deficiency in cats of enzymes needed for glucuronidation (Pascoe et al., 2006). Propofol can cause local pain on injection, which is often worse in small peripheral veins. Some anesthetists will preferentially choose larger veins, administer through a running IV line, or administer a small volume of lidocaine IV immediately before administering propofol to prevent the pain on injection. Propofol without preservative has been associated with increased wound infection. Retrospective analysis of dogs and cats with clean wounds showed an almost four times greater risk of postoperative infection in animals administered propofol (Heldmann et al., 1999). Strict aseptic technique should be emphasized when preparing and administering propofol and unused drug should be discarded within 24 hours (of the nonpreservative formulation). Surgeons performing surgery on patients anesthetized with propofol should be cognizant of this increased risk. Because of the lipid emulsion, propofol has been suggested as a cause of postanesthetic pancreatitis. Pancreatitis associated with propofol has been reported, albeit rarely, in humans; it has not been reported in veterinary species (Gottschling et al., 2005). Furthermore, it is commonly used in patients with active pancreatitis (Herman et al., 2005). Propofol syndrome is a rare but often lethal complication following a prolonged continuous administration of propofol. Patients can develop a metabolic acidosis, rhabdomyolysis, kidney injury, cardiac arrhythmias, and cardiac failure. The pathophysiology of this syndrome appears to involve a disturbance of mitochondrial metabolism induced by propofol (Trampitsch et al., 2006). Neonates and septic patients appear to have a greater risk of developing the syndrome. Any patient on a prolonged infusion of propofol should be monitored for these potential adverse signs. Propofol can be used for short or long-term sedation, induction of general anesthesia, and continuous rate infusions. The induction dose required for Greyhounds is not different from that required for mixed-breed dogs; however, the recovery time was longer for Greyhounds (see Section Metabolism). Propofol can induce oxidative injuries to feline red blood cells when administered as an induction dose followed by a 30 minutes constant rate infusion. This can result in Heinz body formation, anorexia, diarrhea, and malaise (Andress et al., 1995). However, cats administered induction doses of propofol twice daily for 5 days did not show adverse hematological changes (Bley et al., 2007). Propofol should not be used as a sole induction agent in horses as it is associated with excitement. The excitement is likely caused by the long time required to administer the large volume of drug, resulting in the display of stage II anesthesia (excitement stage). Following sedation with an α2-agonist many horses still showed excitement at induction with propofol (Mama et al., 1996). Heavy sedation with guaifenesin has been shown to mitigate the excitement at induction (Brosnan et al., 2011). The quality of recovery from propofol anesthesia horses is good (Mama et al., 1996; Posner et al., 2013). Although good recovery characteristics are important for horses, the presence of excitement during induction and the prohibitive cost for large patients make it unlikely that propofol will be routinely used in equine patients. Propofol does not trigger malignant hyperthermia in susceptible animals (Fruen et al., 1995). However, good sedation is necessary to provide reasonable venous access. Respiratory depression and apnea have been reported with propofol administration in pigs (Tendillo et al., 1996); thus, propofol should be used with caution unless intubation can be readily accomplished. Propofol produces significant respiratory depression in red tailed hawks and great horned owls (Hawkins et al., 2003). It is reasonable to presume that all birds may need ventilatory assistance when anesthesia is maintained with propofol. CNS excitement has been noted at recovery (Hawkins et al., 2003). Propofol has been used to produce anesthesia in fish both intravenously and via immersion (Fleming et al., 2003; Oda et al., 2014). Propofol should be administered slowly to effect. The dose needed will depend on the type and dose of premedication, the health of the patient, and how painful the procedure will be. Premedicants that add CNS depression can decrease induction doses by more than 50%. Time of unconsciousness depends on premedicants and dose of propofol administered. Generally, unconsciousness last from 2–8 minutes. The doses listed in Table 12.3 are provided for the indicated species; however, each patient is different and the total dose should be titrated for a particular patient. Table 12.3 Propofol induction dose and constant rate infusion (CRI) dose for animals Propofol is compatible with 5% dextrose which can be used to dilute propofol for constant rate infusion. Propofol has been mixed with thiopental in a 1 : 1 solution. The mixture has been shown to be stable and provided smooth inductions that were similar to thiopental but better recoveries (more like propofol) (Ko et al., 1999). Accidental overdose of propofol can result in severe cardiovascular and respiratory depression. Treatment is supportive: intubation and ventilation, fluid therapy, positive inotrope, and/or vasoactive drugs. Fortunately, because of rapid recovery, the duration of effect is short. Propofol is a nonscheduled (controlled) drug, but has been proposed to be placed in Schedule IV. Withdrawal times have not been established for animals that produce food. However, FARAD recommends at least 72 hours for meat (Lin and Walz, 2014), but could not offer recommendations for milk due to lack of data. Dissociative anesthetics (e.g., ketamine) have been used extensively in veterinary medicine and may still be the most common class of anesthetics in use. The first dissociative anesthetic used was phencyclidine hydrochloride (PCP, “angel dust”), which is no longer commercially available because of the abuse of this drug among humans. Newer phencyclidine derivatives include ketamine hydrochloride and tiletamine hydrochloride. Ketamine is also a drug of abuse in humans (“special-K”) and tiletamine is only marketed in combination with zolazepam under the trade name Telazol® or Zolatel®. The term dissociative anesthetic originated from the use of ketamine in human patients. When anesthetized with ketamine, patients appeared to feel “dissociated” from or unaware of their environment, but did not always appear asleep (cataleptic). Later it was determined that these drugs did indeed dissociate the thalamocortical and limbic systems causing the change in awareness. Aside from their use as general anesthetics, dissociative anesthetics are used as analgesics for acute and chronic pain states and as well as antidepressants (Stahl, 2013). (Figure 12.3) Ketamine, 2-(o-chloro-phenol)-2-(methylamino)-cyclohexanone hydrochloride, is a phencyclidine derivative that exists as a racemic mixture with the S (+) isomer having greater potency than the R (−) isomer (Casoni et al., 2014). The S (+) isomer produces more analgesia and less emergence delirium than the R (−) isomer. Figure 12.3 Structures of ketamine, tiletamine, and phencyclidine. Tiletamine, 2-[ethylamino]-2-[2-thienyl]-cyclohexan- one hydrochloride, is a phencyclidine derivative. Ketamine is supplied as a 10%, aqueous solution (pH 3.5–5.5). It is preserved with benzethonium chloride. Tiletamine is supplied only in combination with zolazepam as a white powder. The addition of 5 ml diluent produces a solution containing the equivalent of 50 mg tiletamine base, 50 mg zolazepam base, and 57.7 mg mannitol per milliliter. This solution has a pH of 2 to 3.5. Dissociative anesthetics are noncompetitive antagonists at N-methyl-D-aspartate (NMDA) receptors. These drugs prevent the binding of excitatory neurotransmitters, glutamate, and glycine at the NMDA receptor, preventing conduction of ions (Na+, K+, and Ca2+). This prevents firing of the second-order neurons and results in depressed activity at the thalamocortical and limbic systems and depression of nuclei in the reticular activating system. Dissociatives also have weak effects at opioid receptors (mu > kappa), monoaminergic receptors, muscarine receptors, and sigma-1 receptors (endoplasmic reticulum calcium channels), but unlike many other induction agents do not interact with GABA receptors (Annetta et al., 2005; Stahl, 2013). Anesthesia with dissociatives produces an altered consciousness or catalepsy. Unlike other induction agents, dissociatives anesthetics produce analgesia. Analgesia for acute pain is likely at least partially mediated through activation of mu opioids receptors (Sarton et al., 2001). Additionally, the NMDA receptor is also involved in the induction and maintenance of central sensitization in the dorsal horn of the spinal cord (Woolf and Thompson, 1991). Blocking the NMDA receptor with subanesthetic doses of ketamine can interrupt the central sensitization (Correll et al., 2004). Antagonism of the NMDA receptor has also shown great promise in the treatment of refractory depression; however, the mechanism of action as an antidepressant is unknown (Stahl, 2013). Dissociative anesthetics can be used for chemical restraint, rapid induction of general anesthesia, and to provide analgesia (for both acute pain as well as interruption of central sensitization). They can be used in most species and can be administered intramuscularly and intravenously. Based on EEG findings, the antagonism of NMDA receptors produces a dissociation of the thalamocortical and limbic systems (Reich et al., 2005). This produces an altered consciousness or catalepsy, where the patient does not appear asleep, but does not react to noxious or nonnoxious stimuli. Although patient may not look asleep, NMDA antagonists are effective at producing amnesia (Haas and Harper, 1992). When used alone, the dissociative anesthetics rarely produce a surgical depth of anesthesia, but when combined with other CNS depressants produce adequate relaxation and immobility. The CNS effects of dissociatives are different than most other anesthetics. They increase CBF which is coupled with an increase in cerebral glucose metabolism and oxygen demand (CMRO2) (Dawson et al., 1970). The increased CBF is caused by vasodilation of cerebral vessels and an increase in blood pressure, which results in an increase in ICP. Hypercapnia contributes to the rise in ICP and controlling ventilation can attenuate the rise in ICP. Thus, these drugs should be used with caution in any patient having or suspected of having elevated ICP, or where cerebral metabolic demands may not be being met. The effects on ICP are also associated with increased intraocular pressure. Ordinarily, this is not a problem, but these agents should not be used in patients with glaucoma. Ketamine causes the development of EEG patterns that are epileptiform (Kayama, 1982) and is used to elicit more robust seizures during electroconvulsive therapy (Krystal et al., 2003). Interestingly, there is also evidence that ketamine is anticonvulsive (Reder et al., 1980) as well as neuroprotective (Himmelseher and Durieux, 2005). However, most anesthesiologists recommend avoiding ketamine in patients with a seizure history and it should not be used in patients with elevated intracranial pressure. Dissociative anesthetics cause an increase in norepinephrine via central adrenergic stimulation and a decreased reuptake of norepinephrine, resulting in an increase in sympathetic tone (Stoelting, 1999). The increase in norepinephrine affects all systems under adrenergic control (e.g., cardiovascular, respiratory, endocrine, etc.). Emergence delirium (e.g., anxiousness, vocalization, thrashing) is a concern whenever dissociative anesthetics are used. Delirium may be merely unpleasant in smaller animals, but can be dangerous when used in larger animals (e.g., horses). Emergence delirium can be attenuated or prevented by administering dissociative anesthetics with sedatives or tranquilizers (e.g., xylazine, diazepam, acepromazine). The R (+) isomer of ketamine is associated with more delirious effects (Stoelting and Hillier, 2012). Awakening from ketamine is primarily due to redistribution of drug, although some effects are from metabolism. Ketamine has direct myocardial depressant effects (Diaz et al., 1976) but its administration is generally associated with increase in cardiac output, mean aortic pressure, pulmonary arterial pressure, central venous pressure, and heart rate (Haskins et al., 1985). The stimulatory effects are due to directly stimulating the central adrenergic centers (i.e., increased sympathetic tone) and by inhibiting the neuronal uptake of catecholamines, especially norepinephrine (Annetta et al., 2005). The cardiovascular stimulation is associated with an increase in myocardial work and myocardial oxygen demands (Haskins et al., 1985), thus ketamine should be used with caution in patients with cardiovascular disease that cannot tolerate increased metabolic needs. Although the sympathetic stimulation overrides the direct cardiovascular depressant effects of ketamine (Adams, 1997), patients that are catecholamine depleted (i.e., critically ill) may show more of the cardiodepressive effects (Waxman et al., 1980). The increased sympathetic tone also may cause tachycardia. Caution should be used with patients that are already tachycardic or dysrhythmic. Ketamine preserves cardiovascular function and oxygen balance during experimentally produced septic shock (Van der Linden et al., 1990). This cardiovascular sparing effect may be due to the ability of ketamine to block the inflammatory cascade caused by endotoxins that depress cardiovascular function (Taniguchi and Yamamoto, 2005) as well as by increasing levels of norepinephrine that may attenuate the inappropriate peripheral vasodilation seen in septic patients. Dissociative anesthetics generally have a mild effect on minute ventilation and therefore usually cause a moderate increase in carbon dioxide concentrations. This is in contrast to many other anesthetics that are potent respiratory depressants. However, when other CNS depressants are administered with ketamine, significant respiratory depression can be observed. Ketamine also can cause an apneustic pattern of breathing, which is characterized by a rapid sequence of breaths followed by breath holding on inspiration. This occurs more commonly with rapid IV administration of ketamine. Although apneustic breathing is unusual to watch, minute ventilation and carbon dioxide levels are generally within normal limits. Ketamine has bronchodilating properties and decreases airway resistance (Durieux, 1995). This makes it useful as an induction drug for patients with clinical asthma as well as patients with obstructive airway disease (e.g., chronic obstructive pulmonary disease). When ketamine is used as a sole anesthetic, pharyngeal and laryngeal reflexes remain active (Robinson et al., 1986). Preservation of these reflexes, however, leads to an increase risk of laryngospasm and coughing secondary to secretions or manipulation in the oropharynx. These complications make ketamine alone a poor drug for use in endoscopy or oropharyngeal surgery. Although laryngeal reflexes are preserved they can be uncoordinated, and thus aspiration is possible. Endotracheal intubation should be used to maintain and protect the airway. Additionally, ketamine stimulates salivation, which can be copious at times. The administration of an anticholinergic agent (e.g., glycopyrrolate) for its antisialagogue properties can attenuate the amount of salivation. Caution should be exercised when using anticholinergics in patients with small airways as the antisialagogue properties can make for viscous secretions that can occlude the airways or endotracheal tube. Upper airway muscle tone and reflexes are preserved when dissociatives are used alone. However, since these drugs are generally administered with muscle relaxants, laryngeal control is then diminished or absent. Endotracheal intubation is recommended for all anesthetized patients to maintain and protect the airway. Dissociative anesthetics provide little muscle relaxation and may cause muscle rigidity, myoclonus, and/ or uncoordinated muscle movements. Muscle relaxation is generally provided by the coadministration of sedatives or tranquilizers (e.g., benzodiazepine, α2-agonists). The appearance of patients anesthetized with dissociative anesthetics is different than with most other anesthetics. Many patients do not close their eyes, can have muscle tone and muscle movement, and often do not look “asleep”. It is important to protect the corneas of patients with adequate lubrication and prevent objects from rubbing on the cornea. Coadministration of sedatives or tranquilizers can attenuate these signs. As described in the Mechanism of Action section, NMDA antagonists such as ketamine produce analgesia. For many years the dogma has been that ketamine provided greater somatic compared with visceral analgesia. However, this view is too simplistic to explain the varied analgesic effects and should be discarded. The mechanism for antinociception is complex and likely involves interactions at more than one type of receptor. Ketamine provides a use-dependent (i.e., works better when the nerves are stimulated) blockade of the NMDA receptor as well as inhibiting neurotransmitter release, both of which has been shown to mediate nociception (Annetta et al., 2005). Analgesia is also likely mediated by the action of ketamine on mu and kappa opioids receptors (Annetta et al., 2005). Opioid receptors extend beyond the CNS and spinal cord and occur in many peripheral tissues. Ketamine produces analgesia at subanesthetic doses for acute surgical pain and have opioid-sparing effects (Annetta et al., 2005). Additionally, NMDA antagonists at subanesthetic doses are effective at treating chronic pain associated with central sensitization (wind-up pain), neuropathic pain (Guirimand et al., 2000), and likely other types of inflammatory pain. Ketamine by constant rate infusion has been successful in interrupting central sensitization. The duration of the infusion needs to be at least 24 hours, but at times, administration was continued for days to weeks before signs abated (Correll et al., 2004; Sigtermans et al., 2009). When ketamine is used at subanesthetic doses, behavioral changes are rarely seen. Pharmacokinetic values for ketamine in various species are summarized in Table 12.4. Both ketamine and tiletamine are highly lipid soluble, so absorption is rapid from the intramuscular route with peak plasma concentration is reached in ∼10 minutes (Baggot and Blake, 1976). Ketamine can also be absorbed through oral or rectal mucous membranes. This makes it possible to administer by spraying the drug in the mouth of fractious/feral animals or to administer rectally (Hanna et al., 1988). Cats administered ketamine orally often salivate profusely, presumably from the bitter taste or low pH or both. Cats administered ketamine rectally showed a slow smooth induction, no discomfort from this route of administration and a ∼43% availability of drug (Hanna et al., 1988). Table 12.4 Pharmacokinetics of ketamine in various species In most species, ketamine is biotransformed by the liver by N-demethylation to norketamine which then undergoes hydroxylation to form a water-soluble glucuronide derivative that is eliminated in urine (White et al., 1982; Stoelting and Hillier, 2012). Norketamine is an active metabolite of ketamine and has 10–30% activity of the parent drug (Leung and Baillie, 1986; Hanna et al., 1988). In the cat, because of deficiencies in some glucuronidation pathways, ketamine only undergoes transformation to norketamine which is excreted largely unchanged in the urine (Hanna et al., 1988). Since the active metabolites are excreted by the kidneys, decreased renal excretion may prolong the effects of the drug. Telazol is an equal mixture of tiletamine and zolazepam (a benzodiazepine), so the metabolism of this drug needs to be discussed in terms of the two drugs. In cats, the duration of effect of zolazepam exceeds that of tiletamine so that, with time, the pharmacodynamic effect is that of the benzodiazepine tranquilization (Telazol Product-Literature, 2017). In dogs, the duration of effect of tiletamine exceeds that of zolazepam so that, with time, the pharmacodynamic effects reflect the dissociative anesthetic, including muscle rigidity, sympathetic stimulation, and emergence delirium (Telazol Product-Literature, 2017). Other species have similar disparities in metabolism and recoveries from Telazol. Pigs awaken slowly and calmly, whereas horses can have an agitated recovery if not provided with additional sedation. Reversal of the zolazepam in animals with a benzodiazepine antagonist can lead to an anxious recovery if sufficient plasma levels of tiletamine are still present. Ketamine/ tiletamine should not be used in animals with elevated intracranial pressure. Ketamine is a hydrochloride salt, which must be kept at a low pH to maintain solubility. The pH of ketamine is 3.5 which can cause some irritation and pain during IM injections (Hanna et al., 1988). Ketamine/ tiletamine should be used with caution in patients with coronary artery disease, uncontrolled arterial hypertension, cardiomyopathy, or heart failure. Caution should be used in patients where emergence delirium could be injurious to the patient or to medical personnel (e.g., horse). Emergence delirium can be prevented or attenuated by coadministration of sedatives or tranquilizers. In general, dissociative anesthetics should not be administered as sole agents. Ketamine/ tiletamine should not be used animals with elevated intraocular pressure or with an open globe injury due to the rise in intraocular pressure following administration. Ketamine/ tiletamine should be avoided in patients with high sympathetic tone such as hyperthyroidism or pheochromocytoma since administration of these drugs can increase norepinephrine levels and exacerbate the side effects of these diseases. Caution should be used in patients where excretion of the active metabolites may be decreased, such as cats with urethral obstruction or anuric renal failure. Dose ranges for ketamine and tiletamine in various species are listed in Table 12.5. Table 12.5 Dose of ketamine and tiletamine in various species a Following sedation with benzodiazepine, α2-agonist or phenothiazine. b Dose for Telazol is for combination of drugs at 100 mg/ml. c Telazol® should not be used in New Zealand White Rabbits. d TKX = 500 mg Telazol® (as powder) + 250 mg ketamine + 250 mg xylazine. The volume per kg of body weight of Telazol is less than an equipotent amount of ketamine. This makes it attractive for work with zoo/ wildlife animals where the volume may be important (e.g., darts or syringe poles). Horses should not be administered dissociative anesthetics as single agents due to the lack of muscle relaxation and recovery delirium. Telazol in particular can be associated with rough anesthetic recoveries and should be balanced with the use of additional sedatives or tranquilizers, before induction and before recovery. Great controversy occurs over the use of Telazol in the large cats and in tigers specifically. Although there is little published evidence, many anesthesiologists and zoo medicine specialists caution against the use of Telazol in the large cats (Tilson, 1994; Muir et al., 2000; Morris, 2001). Reported complications have ranged from slow ataxic recoveries to delayed (2–4 days after anesthesia) neurological dysfunction, including hind limb weakness, drowsiness, hyperreflexia, hyperresponsive behavior, twitches, seizures, and death (Tilson, 1994; Klein, 2007; Lewis, 2007). At clinical doses, tiletamine (the dissociative part of Telazol) causes lethal renal tubular necrosis in New Zealand White rabbits (Doerning et al., 1992). Other rabbit species appear unaffected. A combination known as TKX (Telazol, ketamine, xylazine) is commonly used to anesthetize pigs. The mixture is composed of 500 mg (as powder) Telazol, 250 mg ketamine, and 250 mg xylazine. Due to slow zolazepam metabolism, pigs can have prolonged recoveries after TKX. Use of ketamine in animals susceptible to malignant hyperthermia has been controversial; however, ketamine did not induce malignant hyperthermia in 76 susceptible pigs (Dershwitz et al., 1989). The concurrent administration of other CNS depressants can increase respiratory and cardiovascular depression. Chloramphenicol can prolong recovery times from ketamine (Amouzadeh et al., 1989) as well as from Telazol. Ketamine has a large therapeutic index; therefore, treatment of an overdose is primarily supportive care and addressing side effect such as respiratory or cardiovascular depression, muscle rigidity, emergence delirium, or adrenergic stimulation. There is no direct antagonist for ketamine or tiletamine. Other sedatives and muscle relaxants are used to manage the problems from an overdose until the drug is cleared. Ketamine extra label use: at least 3 days for meat and 48 hours for milk. Etomidate was first introduced into human anesthesia practice in 1972. At the time it was considered close to the “ideal anesthetic” because of its ability to maintain hemodynamic stability, minimally depress the respiratory centers, and by having a large therapeutic index (i.e., the lethal dose/ effective dose). However, the ideal anesthetic has yet to be found and etomidate too has untoward side effects. Etomidate is a nonscheduled, nonbarbiturate compound suitable for rapid intravenous anesthetic induction. Etomidate is an ethyl ester of a carboxylated imidazole compound with a chemical formula of R-(+)-pentylethyl-1H-imidazole-5 carboxylate sulfate (Figure 12.4). The imidazole component allows for differing solubility at different pH concentrations. At a low (acidic) pH, etomidate is water soluble, but becomes lipid soluble at physiological pH. There are two isomers but only the (R)(+) isomer has anesthetic properties. Figure 12.4 Etomidate. Etomidate is poorly water soluble but highly soluble in alcohol, thus is supplied as a 2 mg/ml solution in 35% propylene glycol at a pH of 6.0. When supplied in propylene glycol, etomidate has an osmolarity of ∼4620 osmole. Etomidate is an agonist at the γ-aminobutyric acid (GABA) receptor (GABAAß3) producing hypnosis and CNS depression by enhancing the effects of the inhibitory neurotransmitter GABA (O’Meara et al., 2004). Binding of GABA to the receptors increases chloride binding, resulting in hyperpolarization of the post synaptic neuron and a decrease in neurotransmission. Etomidate is indicated for the rapid intravenous induction of anesthesia, particularly in patients with cardiovascular instability. Etomidate is considered cerebral friendly because it results in decreased ICP, decreased CBF, and decreased cerebral metabolic rate for O2 (CMRO2). Since the MAP is essentially unchanged, this results in a favorable metabolic state for the CNS; the cerebral perfusion pressure is increased as is the cerebral oxygen supply : demand ratio. The EEG quiets (similar to the barbiturates), but there can be increased EEG activity at epileptogenic foci. Grand mal seizure have been reported, and some anesthetists argue against its use in patients with a known seizure history (Bergen and Smith, 1997). Due to similar mechanisms, the intraocular pressure is also decreased. Intraocular pressure can decrease by up to 60% but the effect lasts for only for ∼5 minutes. Etomidate is unique amongst most intravenous general anesthetics in that it causes minimal hemodynamic changes and thus maintains cardiovascular function. At routine induction doses, etomidate causes minimal changes in stroke volume (SV), MAP, cardiac index (CI), pulmonary artery pressure (PAP), pulmonary arterial wedge pressure (PAWP), central venous pressure (CVP), or systemic vascular resistance (SVR) (De Hert et al., 1990). In addition to causing minimal CV derangements, the baroreceptor and sympathetic nervous system reflexes remain intact, which also contributes to hemodynamic stability. Etomidate is not arrhythmogenic, does not sensitize the heart to catecholamine, and it does not cause histamine release. It is the most commonly used induction agent in dogs having pacemaker implantation (Sanchis-Mora et al., 2014). Etomidate can cause respiratory depression. Induction doses can result in brief periods of apnea, but generally arterial carbon dioxide partial pressure (PaCO2) is only slightly increased if affected at all. Arterial oxygen (PaO2) is generally unaffected. However, when etomidate is administered with other drugs that can affect CNS function, more respiratory depression can be seen. Since etomidate has limited effect on muscle relaxation, airway reflexes are generally maintained. Intubation, however, is highly recommended. In the early 1980s it was noted that there was increased morbidity and mortality in patients sedated long term (e.g., patients on ventilators) with etomidate due to a decreased cortisol production (Wagner et al., 1984). Etomidate causes a reversible inhibition of the enzyme 11-beta-hydroxylase (Figure 12.5), which is an integral part of the pathway that converts cholesterol to glucocorticoids and mineralocorticoids. The resultant decrease in cortisol, corticosterone, and aldosterone production raises concern for patients’ ability to respond to stress. Following induction with etomidate, suppression of the adrenal–cortical axis is depressed for up to 6 hours in dogs and 3 hours in cats (Dodam et al., 1990; Moon, 1997). Single induction doses do not cause clinical problems associated with steroid production, but care should be taken with patients with preexisting adrenal–cortical diseases (e.g., hypoadrenocorticism), or who might have relative hypoadrenocorticism, or adrenal depression from chronic disease. Furthermore, extended periods of use (e.g., constant rate infusions) are not recommended due to suppression of cortisol production (as well as the hyperosmolarity of the solution). Figure 12.5 Pathway for cortisol and aldosterone production affected by action of etomidate. Etomidate does not provide significant muscle relaxation. Muscle rigidity and myoclonus can be seen at, and following, induction but is not associated with seizure-like EEG activity. The use of muscle relaxants (e.g., benzodiazepines) can minimize the incidence and intensity of the muscle movements (Doenicke et al., 1999) and is routinely recommended. Etomidate can potentiate the effects of nondepolarizing neuromuscular blockers. Etomidate does not provide any analgesia. If patients are anesthetized to facilitate surgery or painful procedures, analgesia must be provided separately with other agents (e.g., opioids, α2-agonist). Following intravenous administration, etomidate is rapidly distributed into the CNS to produce anesthesia. Etomidate is 75% bound to plasma proteins. Three compartment pharmacokinetic models have been used to describe etomidate disposition in animals. It has a short redistribution half-life (29 minutes in humans; 22 minutes in cats) and a large steady-state volume of distribution (2.5–4.5 l/kg in humans; 4.9 l/kg in cats). The elimination half-life varies from 2.9 to 5.3 hours in humans and is 2.9 hours in cats (Wertz et al., 1990). Redistribution of etomidate into body tissues is responsible for awakening and recovery time from anesthesia is similar to thiopental, but slower than propofol. In humans, etomidate is 98% metabolized by liver (2% excreted unchanged in urine) by hydrolysis or glucuronidation via plasma esterases and hepatic microsomal enzymes. Metabolites are inactive and excreted in the urine (85%) and bile and feces (15%). Etomidate should be used cautiously in patients with liver disease as metabolism can be prolonged. Adverse effects of adrenocortical suppression and myoclonus have been discussed above. Other adverse effects of etomidate arise from the propylene glycol vehicle. The intravenous injection of propylene glycol can be painful. Pain can be minimized by administering etomidate through a running IV line or by injecting lidocaine (dog) through the catheter before induction. Furthermore propylene glycol causes the solution to be hyperosmotic (∼4620 Osm) compared to plasma (∼300 Osm), and thus etomidate has been associated with intravascular hemolysis (Moon, 1994). Clinically relevant hemolysis has been reported following prolonged administration (i.e., constant rate infusions) in dogs (Ko, 1993; Moon, 1994). Etomidate has been prepared in a lipid vehicle (Etomidate-lipuro, Braun, Germany) instead of propylene glycol, but it is not available in the United States. Etomidate has wide safety margin, due to a large therapeutic index of 16 (i.e., lethal dose is 16 times the hypnotic dose) compared to the therapeutic indexes for propofol and thiopental (3 and 5, respectively). Etomidate should be titrated intravenously “to effect”. The wide dose range reflects the additive and sometimes synergistic effects of many premedications (e.g., opioids, benzodiazepines, phenothiazines). As discussed previously, etomidate should be administered following an adequate premedication. Duration of anesthesia is directly related to dose. Constant rate infusions are not recommended due to the potential for adrenocortical suppression and hemolysis (see Section Adverse Effects/ Contraindications).
Injectable Anesthetic Agents
Indications for Injectable Anesthesia
Disadvantages of Injectable Anesthesia
Properties of an Ideal Injectable Anesthetic Drug
Ideal Physiological Properties
Ideal Pharmacological Properties
Barbiturate Anesthetics
History
Classification
Chemistry:
Drug
Oxygen/ sulfur at position 2
Duration of action
Common use
Phenobarbital
Oxybarbiturate
∼12 h
Anticonvulsant
Pentobarbital
Oxybarbiturate
1–2 h
Anesthesia/euthanasia
Thiopental
Thiobarbiturate
∼20 min
Anesthesia
Thiamylal
Thiobarbiturate
∼20 min
Anesthesia
Methohexital
Oxybarbiturate
∼10–15 min
Anesthesia
How supplied:
Mechanism of Action
Indications
Specific Barbiturates (Box 12.1)
Thiopental sodium (pentothal sodium, previously used name thiopentone sodium):
Thiamylal (Surital):
Pentobarbital sodium (Nembutal sodium):
Euthanasia solutions:
Phenobarbital sodium (Luminal, Phenobarbitone):
Methohexital sodium (Brevital):
Physiological Effects
CNS effects:
Cardiovascular system:
Respiratory effects:
Muscular effects:
Gastrointestinal (GI) tract:
Kidney:
Fetus/neonates:
Miscellaneous:
Analgesia:
Pharmacokinetics
Thiopental:
Phenobarbital:
Phenobarbital:
Metabolism
Adverse Effects/ Contraindications
Dose
Thiopental (mg/kg IV)
Pentobarbital (mg/kg IVa)
Phenobarbital (mg/kg IV)
Methohexital (mg/kg IV)
Dog
8–22
2–30
6.0
3–11
Cat
8–22
2–15
6.0
3–11
Horse
4–15
1.0–10.0
5
Cow
4–22
Sheep
8–15
20–30
Goats
8–20
20–30
Llamas
6–15
Pigs
5–12
10–30
5–8
Mice
30–90 (IP)
Rats
20–40 (IP)
Rabbits
20–50
15–40
10
Chickens
20
Primates
2–20
37
2
Increased risk with clinically ill patients:
Duration of action
Species Differences
Dogs – sighthounds:
Dogs – Greyhounds:
Ruminants:
Swine:
Horses:
Birds:
Reptiles:
Drug Interactions
Overdosage/Acute Toxicity
Propofol
History
Classification
Chemistry:
How supplied:
Mechanism of Action
Indications
Physiological Effects
CNS effects:
Cardiovascular effects:
Respiratory:
Muscular effects:
Miscellaneous:
Pharmacokinetics
Metabolism
Adverse Effects/ Contraindications
Species Differences
Dogs:
Cats:
Equine:
Pigs:
Bird:
Fish:
Dose
Species
Induction dose (mg/kg IV)
CRI dosage (mg/kg/min IV)
Reference
Dog
3–7
0.2–0.6
Cat
5–8
0.5–1.0
Horse – adult
4–8
Mama et al., 1996
Horse – foal
2.0
0.33
Donkey
2
0.21
Pigs
2–3
0.1–0.2
Tendillo et al., 1996
Llama
3.3
0.4
del Alamo, 2014
Ferret
2–4
Rabbit
2–10
Drug Interactions, Compatibilities, and Incompatibilities
Overdosage/acute toxicity:
Regulatory Information
Controlled drug status:
Withdrawal times:
Drug Availability
FDA Veterinary Approved Products
Human Products
Dissociative Anesthetics
History
Classification
Chemistry:
How supplied:
Mechanism of Action
Indications
Physiological/Pharmacodynamic Effects
CNS effects:
Cardiovascular system:
Respiratory:
Muscular effects:
Miscellaneous:
Analgesia:
Pharmacokinetics
Dose
Distribution
Elimination
Protein
Clearance
Species
(mg/kg IV)
phase (min)
half life (min)
binding %
(ml/min/kg)
Reference
Dog
15
1.95
61
53.5
32.2
(Kaka et al., 1979)
Cat
25
2.7
78.7
53
21.3
(Hanna et al., 1988)
Horse
2.2
2.9
42
50
26.6
(Kaka et al., 1979)
Calf
5.0
6.9
60.5
40.4
(Waterman, 1984)
Humans
2.2
4.68
130
60
(Domino et al., 1984)
Metabolism
Ketamine:
Telazol:
Adverse Effects/ Contraindications
CNS disease:
Pain from injection:
Cardiovascular disease:
Emergence delirium:
Ocular disease:
Increased sympathetic tone:
Urethral obstruction or renal insufficiency:
Dose
Species
Dosage of ketamine (mg/kg)a
Dosage of Telazol®(mg/kg) b
Dog
2–5 IV, 5–10 IM
3–6 IM
Cat
5–10 IV, 5–10 IM
10–20 PO
25 rectally
4–7 IM
Cattle
2–4 IV
2–4 IV
Camelid
2–4 IV
0.5–2 IV, 2–4 IM
Ferret
2–5 IV, 5–10 IM
Horses
2 IV
1–3 IV (following α2-agonist)
Rat
40–80 IM
30–60
Pigs
10–30 IM
6 IM (following α2-agonist)
0.01–0.02 ml/kg as TKXd
Rabbits
20–50 IM
30–60 IMc
Primates
2–4 IV, 10–15 IM
3 IM
Species Differences
Horse:
Tigers:
Rabbits:
Pigs:
Drug Interactions
Overdosage/Acute Toxicity
Regulatory Information
Withdrawal time:
Controlled drug schedule:
Drug Availability
FDA Veterinary Approved Products
Human Approved Products
Etomidate
History
Classification
Chemistry:
How supplied:
Mechanism of Action
Indications
Physiological Effects
CNS effects:
Cardiovascular system:
Respiratory:
Endocrine system:
Muscular effects:
Analgesia:
Pharmacokinetics
Metabolism
Adverse Effects/ Contraindications
Dose (Box 12.2)
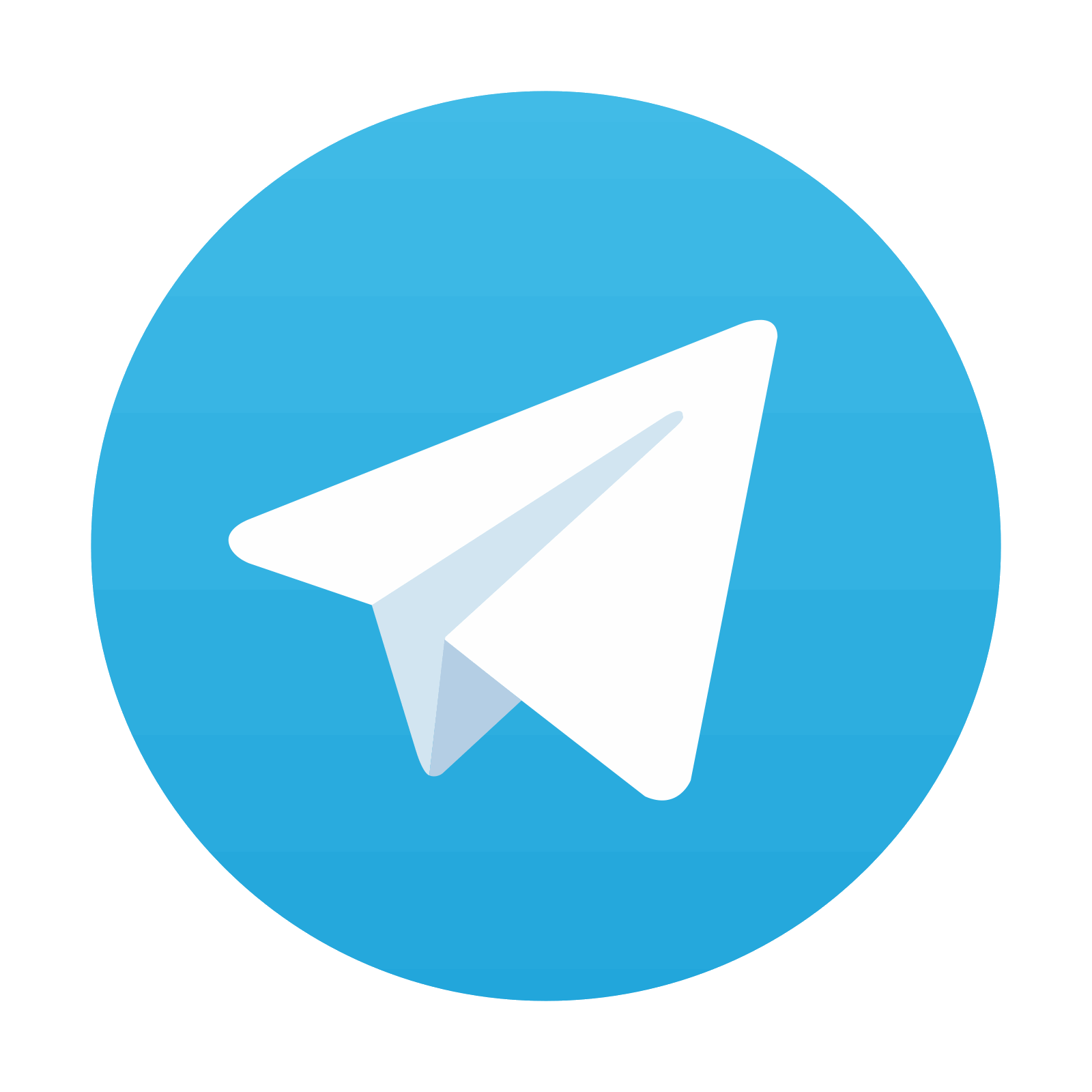
Stay updated, free articles. Join our Telegram channel
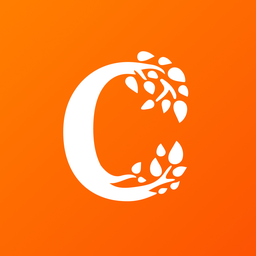
Full access? Get Clinical Tree
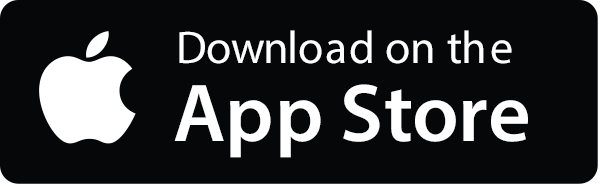
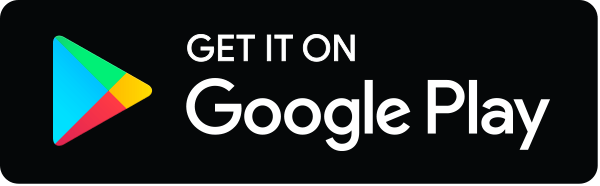