11 Robert J. Brosnan and Eugene P. Steffey Inhalation anesthetics are unique among the anesthetic drugs because they are administered, and in large part removed from the body, via the lungs. They are used widely for the anesthetic management of animals in part because their pharmacokinetic characteristics favor predictable and rapid adjustment of anesthetic depth. In addition, a special apparatus is usually used to deliver the inhaled agents. This helps minimize patient morbidity or mortality because it facilitates accurate and controlled anesthetic delivery, lung ventilation, and improved arterial oxygenation. Partial pressures of modern agents also rapidly equilibrate between the lung alveoli and active sites within the central nervous system (CNS). Breath-by-breath monitoring of end-tidal (alveolar) anesthetic concentrations can be routinely monitored by agent analyzers that, in effect, allow indirect breath-by-breath monitoring of drug partial pressures within CNS sites responsible for anesthetic action. Over the nearly 170 years that inhalation anesthesia has been used in clinical practice, fewer than 20 agents have actually been introduced and approved for general use with patients. Fewer than 10 of these have had any history of widespread clinical use in veterinary medicine, and only four are currently of major clinical importance in North America (Table 11.1). All are relatively old agents, the most recent – sevoflurane – having been first synthesized and studied in the 1970s (Terrell, 2008). However, the reason for this apparent lack of drug innovation is not because the ideal inhalation anesthetic has already been identified. An ideal agent would have characteristics that include a stable shelf life without preservatives and compatibility with existing delivery equipment. It would be inexpensive to purchase, nonflammable, and easily vaporized under ambient conditions and have a high boiling point. Such an agent would have a low blood solubility to foster rapid changes in anesthetic depth and permit rapid, controlled recovery from anesthesia. The ideal agent would exhibit potency sufficient to produce surgical anesthetic planes at concentrations less than 50–60% of one atmosphere partial pressure. There would be no cardiopulmonary depression or cardiac sensitization to arrhythmias; the agent would not be irritating to airways and would have a pleasant odor (or be odorless). The drug would not lower seizure threshold, would not cause neuronal injury, and would provide analgesia (or at least not cause hyperalgesia). Finally, it would produce good skeletal muscle relaxation, resist degradation in the anesthetic delivery apparatus, undergo no biotransformation, be nontoxic to the kidneys and liver, and not cause emesis. No inhalation agent in current use fulfills all of these criteria. Table 11.1 Inhalation anesthetic agents used in animals in North America The chemical structure of inhalation anesthetics and their physical properties are important to their actions and determine how these drugs are administered, what delivery and scavenge equipment is required, which techniques can be used to monitor vapor concentrations in patients, and whether or how these agents are metabolized. A large number of structurally disparate agents have been delivered by inhalation to produce general anesthesia, including single atom elements (Lawrence et al., 1946a; Koblin et al., 1998), diatomic elements (Koblin et al., 1998), oxides of carbon (Thompson, 1912; Brosnan et al., 2007) and nitrogen (Wells, 1847), cyclic alkanes (Waters and Schmidt, 1934), unhalogenated (Taheri et al., 1993; Liu et al., 1994) and halogenated (Snow, 1858; Suckling, 1957; Whalen et al., 2005) n-alkanes, unhalogenated (Riggs, 1925) and halogenated (Hewer, 1942; Dingman and Lim, 1963) alkenes, unhalogenated (Jackson and Morton, 1847; Krantz et al., 1946) and halogenated (Artusio et al., 1960; Terrell, 2008) ethers, among others. With the exception of the inorganic compound nitrous oxide (N2O), contemporary anesthetics in common use are either halogenated ethers or a halogenated alkane (Figure 11.1). Halogenation renders modern organic anesthetics nonflammable at clinically relevant concentrations. Figure 11.1 Chemical structures of inhalation anesthetics of clinical (halothane, methoxyflurane, enflurane, isoflurane, sevoflurane, desflurane), investigational (xenon), and historical (diethyl ether, chloroform) interest. Clinical administration of inhalation anesthetics requires a carrier gas that must include oxygen, a source of anesthetic, and a patient breathing circuit. For very small animals (e.g., laboratory rodents or small birds) this may mean nothing more than placing the animal in a closed air-filled chamber that contains a cotton pledget saturated with liquid anesthetic (e.g., isoflurane). With larger animals and/or to provide more controlled delivery of anesthetic and O2, it is more appropriate to use a specialized delivery apparatus to improves the safety and control of anesthetic concentrations delivered to patients. The anesthetic machine includes one or more flow meters, one or more vaporizers, and a patient breathing circuit. Reviews of vaporizers and other anesthetic delivery equipment are available elsewhere (Dorsch and Dorsch, 2007; Tranquilli et al., 2007; Miller et al., 2014). Inhalation anesthetics are either gases or vapors. N2O exists as a gas under operating room conditions because at 1 atmosphere pressure, the boiling point of N2O is −89°C. Consequently, N2O for clinical use in tanks is compressed to the liquid state at about 750 psi (pounds per square inch; 750 psi/14.9 psi [1 atmosphere] = 50 atmospheres). As the N2O gas is drawn from the tank, liquid N2O is vaporized, and the overriding gas pressure remains constant until no further liquid remains in the tank. At that point, only N2O gas remains, and the gas pressure decreases from this point as remaining gas is vented from the tank. The term “vapor” indicates the gaseous state of a substance that at ambient temperature and pressure is a liquid; volatile anesthetics have sufficient vapor pressure to be administered via inhalation. The volatile agent desflurane has a boiling point of 23.5°C (Table 11.2); it boils (becomes a gas) only slightly above typical 20°C ambient room temperature or at altitudes higher than 1100 meters (3600 feet) due to decreased barometric pressure. As a result, a specialized vaporizer is required to maintain temperature and pressure constant and allow for precise and safe delivery of this agent. Table 11.2 Some physiochemical properties of volatile anesthetics. Source: Data from Lerman et al., 1983; Taheri et al., 1991; Soares et al., 2012. Quantities of inhalation anesthetic agent are usually characterized by one of three methods: pressure (e.g., in mmHg), concentration (in volumes), or mass (in mg or g). The form most familiar to clinicians is that of concentration (e.g., X% of agent A in relation to the whole gas mixture). Modern monitoring equipment samples inspired and expired gases and provides concentration readings for inhalation anesthetics. Precision vaporizers used to control delivery of inhalation anesthetics are calibrated in percentage of agent, and effective doses are almost always reported in percentages. Finally, the molecular weight and agent density are used in ideal gas calculations to convert from liquid to vapor volumes and mass (Hill, 1980). The vapor pressure of an anesthetic is an indicator of its ability to evaporate; it reflects the tendency for molecules in the liquid state to enter the gaseous (vapor) form. The vapor pressure of a potent volatile anesthetic must be sufficient to provide enough molecules of anesthetic in the vapor state to produce anesthesia at ambient conditions. The saturated vapor pressure represents a maximum concentration of molecules in the vapor state that can exist for a given liquid at each temperature. The saturated vapor concentration can be easily determined by relating the vapor pressure to the ambient pressure. Using halothane and associated information from Table 11.2 as an example, we see that a maximal concentration of 32% halothane is possible under usual operating-room conditions; that is, 244/760 × 100 = 32%, where 244 mmHg is the vapor pressure at 20°C and 760 mmHg is the barometric pressure at sea level. Thus, other variables considered constant, the greater the vapor pressure, the greater the concentration of the drug deliverable to the patient. As described by Henry’s Law, the partial pressure exerted by a concentration of gas in solution is inversely proportional to the solubility of that gas in that solvent. The solubility of an anesthetic is a major characteristic of the agent and has important clinical ramifications. For example, anesthetic solubility in blood and body tissues is a primary factor in the rate of uptake and distribution within the body. It is therefore a primary determinant of the speed of anesthetic induction and recovery. Solubility in lipid bears a strong relationship to anesthetic potency, and the tendency to dissolve in anesthetic delivery components such as rubber influences equipment selection and other aspects of anesthetic management. The extent to which a gas will dissolve in a given solvent is usually expressed in terms of its partition coefficient (PC)(Table 11.3), a distribution constant that describes the concentration ratio of a gas between two bulk phases (e.g., between blood and gas) at a specified temperature. It thus describes the affinity or capacity or solubility of an anesthetic for one solvent phase relative to another, that is, how the anesthetic will partition itself between two phases after equilibrium has been reached. Anesthetic gas movement occurs because of a partial pressure difference in the two phases so that when there is no longer any anesthetic partial pressure difference between the two phases, there is no longer any net movement of anesthetic in either phase direction, and equilibrium has been achieved. Solvent/gas and human tissue/gas PCs are summarized in Table 11.3 ; some of these differ significantly from values in animals for some agents (Soares et al., 2012). Table 11.3 Partition coefficients at 37°C. Source: Data from Eger, 1981; Goto et al., 1998; Lynch et al., 2000; Eger et al., 2003a. Of all the PCs that have been described or are of possible interest, two are of particular importance in the practical understanding of anesthetic management. They are the blood/gas and the oil/gas partition coefficients. The blood/gas solubility (Table 11.3) is a determinant of the speed of anesthetic onset (induction or change in anesthetic level) and offset (recovery). All else being equal, a lower blood/gas PC allows for more rapid anesthetic induction or rate of change of anesthetic level in response to a stepwise change in anesthetic delivery. The oil/gas PC is another solubility characteristic of clinical importance (Table 11.3). This PC describes the ratio of concentration of an anesthetic in oil (in this case olive oil is the generally agreed upon standard) and gas phases at equilibrium. The oil/gas PC correlates inversely with anesthetic potency and describes the capacity of lipids for anesthetic. Solubility characteristics for tissues (Table 11.3) and other media like rubber and plastic (components of anesthetic delivery equipment; Table 11.4) are also important. For example, tissue solubility determines in part the quantity of anesthetic removed from the blood to which it is exposed. The higher the tissue solubility, the more anesthetic is removed from the blood, which slows the rate of rise of anesthetic partial pressure at active sites. During recovery, anesthetic can leave these tissue reservoirs and oppose anesthetic washout and delay recovery time. High agent solubility in rubber (Table 11.4) will oppose the rise of anesthetic partial pressure within the anesthetic apparatus (such as in an equine anesthetic circuit that often uses a substantial quantity of rubber in hoses and breathing bags) and slow anesthetic delivery to the patient. Table 11.4 Rubber or plastic/gas partition coefficients at room temperature. Source: Data from Marx et al., 1996; Eger et al., 2003a. The aim in administering an inhalation anesthetic to a patient is to achieve partial pressure or tension of anesthetic (Panes) in the CNS to achieve a desired level of anesthesia. Movement of molecules of inhalation anesthetics, like O2 and CO2, occurs down partial pressure gradients (Figure 11.2). Gases move from regions of higher tension to those of lower tension until equilibrium (i.e., equal partial pressure in the two media) is established. Anesthetic partial pressure is highest immediately downstream from the vaporizer and is progressively less as gas travels to a semiclosed anesthetic breathing circuit, from circuit to lungs, from lungs to arterial blood, and, finally, from arterial blood to body tissues such as the brain and spinal cord (Figure 11.2). With modern drugs, the anesthetic partial pressure in the pulmonary alveoli rapidly equilibrates the anesthetic partial pressure in highly vascular tissues receiving large arterial blood flow (such as sites of anesthetic action within the CNS). Hence, the alveolar partial pressure (PA) of anesthetic is in a delayed equilibrium with the CNS partial pressure of anesthetic. Monitoring drug alveolar partial pressure allows indirect real-time monitoring of the effect site concentration. Figure 11.2 The movement of inhaled anesthetic agents down a series of partial pressure gradients (gas tension gradients) during induction and recovery. During induction there is a high anesthetic tension in the vaporizer that decreases progressively towards the site of action in the CNS. The PA of anesthetic is a balance between anesthetic input (i.e., delivery to the alveoli) and loss (uptake by blood and body tissues) from the lungs (Figure 11.2). A rapid rise in PA of anesthetic is associated with a rapid anesthetic induction or change in anesthetic depth. Factors that contribute to a rapid change in PA of anesthetic are summarized in Table 11.5. Table 11.5 Factors that promote a rapid change in alveolar anesthetic tension Delivery of anesthetic to the alveoli – and the rate of rise of the alveolar concentration or fraction (FA) toward the inspired concentration or fraction (FI) – depends on the inspired anesthetic concentration itself and the magnitude of alveolar ventilation. Increasing either one of these or both increases the rate of rise of the PA of anesthetic. The upper limit of inspired concentration is dictated by the agent vapor pressure at a given temperature. Characteristics of the patient breathing system are also important, including the type of circuit (open, semiopen, semiclosed, or closed), the amount of rubber or plastic in the system, the position of the vaporizer relative to the breathing circuit (i.e., within or outside the circuit), and the fresh gas inflow to the patient breathing circuit (Eger, 1974; Dorsch and Dorsch, 2007; Tranquilli et al., 2007; Brosnan, 2013; Miller et al., 2014). An increase in alveolar ventilation increases the rate of delivery of inhalation anesthetic to the alveoli. If unopposed, alveolar ventilation would rapidly increase the alveolar concentration of anesthetic so that within minutes the alveolar concentration would equal the inspired concentration. However, in reality, the input created by alveolar ventilation is initially diluted by the volume of the lung’s functional residual capacity (FRC) and subsequently by absorption of anesthetic into blood. Hypoventilation reduces the rate at which the alveolar concentration increases over time compared to the inspired concentration. Anesthetic uptake is the product of three factors: solubility (S; the blood/gas solubility, Table 11.3), cardiac output (CO), and the difference in the anesthetic partial pressure between the alveoli and venous blood returning to the lungs (PA–Pv; expressed in mmHg); that is, where Pbar = barometric pressure in mmHg (Eger, 1974). Note that if any of these three factors equals zero, there is no further uptake of anesthetic by blood. The solubility of an inhalation anesthetic in blood and tissues is characterized by its partition coefficient (PC; Table 11.3). Compared to an anesthetic agent with high blood solubility (PC), an agent with low blood solubility should be associated with a more rapid equilibration between tissue phases because a large amount of the highly soluble anesthetic must be dissolved in the blood before equilibrium is reached with the gas phase. In the case of the agent with a high blood/gas PC, the blood acts like a large “sink” into which the anesthetic is poured, and thus a large blood anesthetic concentration is required before a large blood anesthetic partial pressure can be reached. It is the partial pressure gradient, and not the concentration gradient, that determines diffusion of gases. As a result, it takes more soluble anesthetics more time to reach a given blood partial pressure and CNS partial pressure than less soluble anesthetics. Anesthetic agents with a low blood/gas PC are desirable because they permit: (i) a more rapid anesthetic induction (i.e., more rapid rate of rise in alveolar concentration during induction; Figure 11.3); (ii) more precise control of anesthetic depth (i.e., alveolar concentration during the anesthetic maintenance phase of anesthesia); and (iii) a more rapid elimination of anesthetic and recovery from anesthesia (i.e., a rapid decrease in alveolar concentration during the anesthetic recovery phase). Figure 11.3 The rise in the alveolar (FA) anesthetic concentration toward the inspired (FI) concentration in humans. Note the rise is most rapid with the least soluble anesthetic, N2O, and slowest with the most soluble anesthetic, methoxyflurane. Source: Data from Eger and Eger, 1985; Eger, 1992. Cardiac output, the amount of blood flowing through the lungs (or systemic circulation) per unit time, influences anesthetic uptake from the lungs. With greater cardiac output, there is a greater quantity of blood passing through the lungs that will take up anesthetic from the alveoli. A large CO, like increased anesthetic agent blood solubility, delays the alveolar rise of Panes and anesthetic onset. The magnitude of difference in anesthetic partial pressure between the alveoli and venous blood is related to the amount of uptake of anesthetic agent by tissues. Not surprisingly, the largest gradient occurs during induction. Once the tissues no longer absorb anesthetic (i.e., equilibrium between the two phases is reached), there is no longer any uptake of anesthetic agent from the lungs; the venous blood returning to the lungs contains as much agent as when it left the lungs. The changes in gradient in between these extremes result from the relative distribution of CO. In this regard it is important to recognize that roughly 70–80% of the CO is normally directed to only a small volume of body tissues in a lean individual (Table 11.6) (Eger, 1974; Staddon et al., 1979; Yasuda et al., 1990; Eger and Saidman, 2005). Tissues such as brain, heart, hepatoportal system, and kidneys represent only about 10% of the body mass but normally receive about 75% of the total blood flow each minute. These highly perfused tissues equilibrate relatively rapidly with the arterial anesthetic tension. Since the venous anesthetic tension equals that in the tissue within 10–15 minutes, about 75% of the blood returning to the lungs is the same as the alveolar tension, presuming that arterial anesthetic tension is held constant. Thus anesthetic uptake is reduced over time. Skin and muscle compose the major bulk of the body (about 50% in humans) but at rest receive only about 15–20% of the CO, so saturation of this tissue group may require up to a few hours to accomplish. Fat is a variable component of body bulk and receives only a small proportion of blood flow. Consequently, anesthetic saturation of this tissue group is very slow, especially given that all anesthetics are considerably more soluble in fat than in other tissue groups (Table 11.3). Table 11.6 Blood flow characteristics in different tissue groups. Source: Eger, 1974. Reproduced with permission of EI Eger. aBrain, heart, hepatoportal, kidney are examples of components of the “vessel rich” grouping and ligaments, tendons, bone are examples within the “vessel poor” tissue grouping. Other factors can further influence the magnitude of the alveolar-to-arterial anesthetic partial pressure gradient. They include body size (Wahrenbrock et al., 1974), abnormalities of lung ventilation/perfusion (Eger and Severinghaus, 1964), loss of anesthetic via the skin (Stoelting and Eger, 1969b; Fassoulaki et al., 1991; Lockhart et al., 1991b) and into closed gas spaces (Eger, 1974; Eger, 1985; Eger et al., 2003a), and metabolism (Eger, 1974; Eger et al., 2002; Eger et al., 2003a). Recovery from inhalation anesthesia is a consequence of anesthetic elimination from the CNS. This first requires washout of anesthetic from the alveoli and blood (Figure 11.2). Factors favoring a faster rate of rise of CNS anesthetic partial pressure are the same factors that favor faster anesthetic elimination: alveolar ventilation, CO, and the agent blood/gas partition coefficient. Indeed, graphs representing anesthetic wash-out from the alveoli versus time (Figure 11.4) are essentially inverse of the anesthetic wash-in curves (Figure 11.3). Figure 11.4 The fall in alveolar (FA) concentration relative to the alveolar concentration at the end of anesthesia (FA0). Note that the newest, most insoluble volatile anesthetic, desflurane, is eliminated in humans more rapidly than the other contemporary potent anesthetics. Not shown is information for methoxyflurane; if present the curve for methoxyflurane would appear above that for halothane. Source: Eger, 1992. Reproduced with permission of Wolters Kluwer Health. Anesthetic elimination kinetics also exhibit context sensitivity with respect to time; drug washout is slower as the duration of anesthesia increases. The relative context sensitivity increases as a function of the agent solubility in blood and body tissues (Eger and Johnson, 1987; Eger, 1993; Eger et al., 1998; Eger and Saidman, 2005; Eger and Shafer, 2005). During anesthetic maintenance, agent concentrations gradually increase in less perfused muscle and viscera and, more slowly, in fat. These large tissue volumes become an ever increasing reservoir of anesthetic until their partial pressure equilibrates with that of the arterial blood. During recovery, gradual anesthetic washout from less vascular tissues can oppose the fall in anesthetic partial pressure in the blood and redistribute to the CNS, thereby delaying anesthetic recovery. Other factors contributing to anesthetic elimination from the body include percutaneous loss (Stoelting and Eger, 1969b; Cullen and Eger, 1972; Fassoulaki et al., 1991; Lockhart et al., 1991b), intratissue (Neumann et al., 2005) or intertissue (Carpenter et al., 1986b; Carpenter et al., 1987) diffusion of agents, and body size and composition (Lemmens et al., 2008). Metabolism plays a small role with some older inhalation anesthetic agents, such as methoxyflurane or halothane (Cahalan et al., 1981; Carpenter et al., 1986a; Carpenter et al., 1987), but not with newer volatile agents which are largely eliminated unchanged by the respiratory system. The standard index of potency for inhalation anesthetics is the minimum alveolar concentration (MAC) (Merkel and Eger, 1963; Eger et al., 1965; Eger, 2002), the alveolar concentration of an inhaled anesthetic that prevents gross nonreflexive movement in 50% of subjects exposed to a supramaximal noxious stimulus. Thus, MAC is equivalent to the median effective dose, or ED50, for inhaled anesthetics. The dose that corresponds to the ED95 (95% of the individuals are anesthetized), at least in humans, is 20–40% greater than MAC (de Jong and Eger, 1975); and 2.0 times the MAC (i.e., 2 MAC) represents a deep level of anesthesia, in some cases even an anesthetic overdose. MAC values for contemporary inhalation anesthetics in select animal species are summarized in Table 11.7. Table 11.7 The minimum alveolar concentration (MAC) of inhalation anesthetics. Source: Data from Lynch et al., 2000; Steffey and Mama, 2007. The anesthetic potency of an inhaled anesthetic is inversely related to MAC (i.e., potency = 1/MAC). MAC of conventional anesthetics is inversely related to the oil/gas PC, as described by the Meyer–Overton relationship (Figure 11.5) (Meyer, 1899; Overton, 1991). Thus, a very potent anesthetic (e.g., methoxyflurane) has a low MAC value and a high oil/gas PC; an agent of low anesthetic potency (e.g., N2O) has a high MAC and a low oil/gas PC. Figure 11.5 Oil/gas partition coefficients versus potency (MAC) of inhalation anesthetics in dogs, humans and mice. Source: Miller et al., 2014. Reproduced with permission of Elsevier. It is important to note that MAC is normally determined in healthy animals under controlled laboratory conditions in the absence of other drugs and other circumstances common to clinical use that may modify anesthetic requirements. Many factors may either increase or decrease MAC (anesthetic requirement). The interested reader is referred elsewhere for reviews of this topic (Stoelting and Hillier, 2006; Tranquilli et al., 2007; Miller et al., 2014). By virtue of their effects on multiple cellular targets, inhaled anesthetics have widely distributed and largely depressant effects on function of most body systems. Though the magnitude of some actions vary with agent, the effect profile of most potent modern alkane and ether type anesthetics is remarkably similar. In contrast, the less potent inorganic anesthetics (N2O and Xe) may not produce similar anesthetic endpoints and side effects in all species at standard pressures. Following is an overview of major inhalation anesthetic effects on body systems with a focus on drug effects in healthy patients. However, the reader is cautioned that effects both desirable (e.g., immobilization potency) and adverse (e.g., cardiorespiratory depression) may be modified by a number of variables encountered in clinical and laboratory settings, including species, genetics, duration of anesthesia, noxious (surgical) stimulation, ventilation mode, coexisting disease, and concurrent medications. We refer the reader to additional references that discuss these interactions in greater detail (Tranquilli et al., 2007; Miller et al., 2014). Inhalation anesthetics produce a reversible generalized CNS depression for which the degree of depression is often described as depth of anesthesia. The components of general anesthesia have traditionally been considered to include: unconsciousness, amnesia, analgesia, and immobility. Some also include in this concept, suppression of autonomic reflexes. Most recently, in attempting to better define the site(s) and mechanism(s) of action of inhalation anesthetics this list has been distilled to just two reversible qualities applying to all inhalation agents: amnesia and immobility in response to a noxious stimulus (Eger et al., 2003a; Eger and Sonner, 2006). The inhaled agents cause immobility and amnesia, but these distinct endpoints are achieved via actions at different CNS sites. In an experimental goat model in which the cerebral circulation was isolated from the rest of the body, selective brain isoflurane administration more than doubled the anesthetic requirement for immobility compared to whole-body isoflurane administration. This demonstrated that the spinal cord, and not the brain, is principally responsible for preventing movement during surgery with inhaled anesthetics (Antognini and Schwartz, 1993). Within the spinal cord, immobility is most likely produced by depression of locomotor neuronal networks located in the ventral horn (Jinks et al., 2005). In contrast, amnestic effects of inhaled anesthetics are produced by actions within the brain, most probably within the amygdala and hippocampus (Eger et al., 2003b). Lesions created within the amygdala of rats can block amnestic actions of sevoflurane (Alkire and Nathan, 2005). On electroencephalography, hippocampal-dependent θ-rhythm frequency slows in proportion to the amnestic effects observed with subanesthetic concentrations of isoflurane in rats (Perouansky et al., 2010). Furthermore, mutant mice lacking the gene encoding either the α4 or β3 subunits of GABAA receptors are resistant to isoflurane depression of hippocampus-dependent learning and memory (Rau et al., 2009; Rau et al., 2011), and antagonism of α5 subunit-containing GABAA receptors restores hippocampal-dependent memory during sevoflurane administration (Zurek et al., 2012). Clearly the mechanisms of inhaled anesthetic action responsible for amnesia are different from those responsible for immobility. At concentrations above MAC, it is presumed that inhaled anesthetics sufficiently depress cortical function to prevent animals from experiencing motivational-affective dimensions of pain (Melzack and Casey, 1967). Additionally, concentrations of modern volatile agents (halothane, enflurane, isoflurane, sevoflurane, desflurane) between 0.8 and 1.0 times MAC decrease (but do not ablate) wind-up and central sensitization and so may help prevent heightened postoperative pain sensitivity; however, higher concentrations of contemporary drugs offer no further benefit in this regard (O’Connor and Abram, 1995; Mitsuyo et al., 2006). Volatile anesthetic concentrations between 0.4 and 0.8 times MAC decrease withdrawal responses to noxious stimuli, but lower concentrations can actually cause hyperalgesia with a peak effect at 0.1 times MAC (Sonner et al., 1998; Zhang et al., 2000) due to potent nicotinic cholinergic receptor inhibition (Flood et al., 2002). In contrast, the gaseous anesthetics xenon and nitrous oxide produce analgesia via glutamatergic receptor inhibition (Yamakura and Harris, 2000), and in the case of nitrous oxide, through additional modulation of noradrenergic–opioid receptor pathways (Zhang et al., 1999). Anatomically, analgesic actions occur both supraspinally and within the dorsal horn of the spinal cord, and are likely responsible for greater blunting of autonomic responses associated with these gases as compared to the modern volatile agents (Nakata et al., 1999). Unconsciousness, at least insofar as it may be correlated with loss of the righting reflex and spontaneous movement (Quasha et al., 1980), may occur at yet other sites within the CNS. Nicotine injections into the central medial thalamus causes partial-to-full arousal in rats lightly anesthetized with sevoflurane, suggesting a role of nicotinic acetylcholine receptors within this region (Alkire et al., 2007). Within the midbrain, electrical stimulation (Solt et al., 2014) or injection of D1 receptor agonists (Taylor et al., 2013) into the ventral tegmental area reverses the immobilizing effects of isoflurane in rats, thus indicating a role for dopamine receptors. Presumably, thalamic and brainstem arousal allows subsequent activation of other cortical regions responsible for information processing and integration, a hallmark of consciousness (Tononi, 2008). Of the contemporary inhaled anesthetics, enflurane (Joas et al., 1971; Neigh et al., 1971) and sevoflurane (Adachi et al., 1992; Komatsu et al., 1994; Osawa et al., 1994; Woodforth et al., 1997; Hilty and Drummond, 2000) are capable of producing epileptiform activity during electroencephalography. However, tonic–clonic muscle activity is typically only observed with enflurane, whereas sevoflurane may actually raise the threshold for seizure manifestation (Karasawa, 1991; Fukuda et al., 1996; Murao et al., 2000a), although perhaps less so than other inhaled agents (Murao et al., 2002). Isoflurane (Koblin et al., 1981; Fukuda et al., 1996; Murao et al., 2000a, 2000b) and desflurane (Fang et al., 1997b) suppress convulsive activity induced by such drugs as bupivacaine, lidocaine, and penicillin. Inhalation anesthetics are cerebral vasodilators and tend to increase cerebral blood flow and interfere with normal cerebral vascular autoregulation. These effects are agent dependent (less with sevoflurane) (Conzen et al., 1992; Gupta et al., 1997; Matta et al., 1999; Nishiyama et al., 1999; Summors et al., 1999; Bedforth et al., 2000), dose dependent (less at sub-MAC concentrations) (Bundgaard et al., 1998; Matta et al., 1999; Werner et al., 2005), and species dependent (autoregulation better preserved in horses than humans and small animals) (Brosnan et al., 2011). Cerebrovasodilation increases intracranial blood volume and pressure, resulting in increased intracranial pressure that is exacerbated by head-down body positioning (Brosnan et al., 2002a, 2002b), hypercapnia (Brosnan et al., 2003a, 2003b), prolonged anesthetic duration (Brosnan et al., 2003a), and pathophysiological changes associated with increased intracranial elastance (Brosnan et al., 2004), such as intracranial masses, hemorrhage, edema, and hydrocephalus (North and Reilly, 1990). Severe and uncompensated increases in intracranial pressure can cause cerebral herniation and also decrease the extracranial-to-intracranial pressure gradient, defined as the cerebral perfusion pressure, resulting in cerebral ischemia. In the adult, inhaled anesthetic preconditioning offers some degree of neuroprotection during subsequent hypoxic or ischemic insults (Xiong et al., 2003; Kehl et al., 2004; Zheng and Zuo, 2004; Payne et al., 2005; Li et al., 2008; Li and Zuo, 2009; Yang et al., 2011). However, in neonates, many inhaled agents can adversely affect neural viability or neural development. Contemporary volatile anesthetics have been associated with neuronal apoptosis in the brains and spinal cords of neonatal rats (Sanders et al., 2008b; Istaphanous et al., 2011) and monkeys (Brambrink et al., 2010, 2012); the longer-term clinical consequences for behavioral changes or learning and memory deficits are presently unresolved (Jevtovic-Todorovic et al., 2003b; Culley et al., 2004; Loepke et al., 2009; Satomoto et al., 2009; Kodama et al., 2011). In the aged, inhaled agents have been associated with postanesthetic cognitive dysfunction (Culley et al., 2003; Zhang et al., 2012) with the suggestion that impairment could be secondary to accumulation of amyloid protein within the brain (Xie et al., 2006). Although important in animals, implications of these potential side effects are even greater for humans. All contemporary inhalation anesthetics depress alveolar ventilation. As a consequence, the PaCO2 is increased in direct relation to anesthetic dose (MAC multiple; Figure 11.6). The magnitude of PaCO2 is also species related (Figure 11.7). In addition, the normal stimulation to ventilation caused by an increased PaCO2 and/or a low arterial oxygen tension (PaO2) is reduced (Knill and Gelb, 1978; Knill et al., 1983; Dahan et al., 1994, 1996; Sarton et al., 1996; Sjogren et al., 1998, 1999; Miller et al., 2014). Figure 11.6 A summary of the effects of contemporary volatile anesthetics on PaCO2 in humans. Source: Data from Munson et al., 1966; Larson et al., 1969; Calverley et al., 1978; Doi and Ikeda, 1987a; Lockhart et al., 1991a. Figure 11.7 The PaCO2 (mean ± SE; mm Hg) in spontaneously breathing, health dogs, horses, humans and monkeys during halothane-oxygen anesthesia. The anesthetic dose is expressed as a multiple of the minimum alveolar concentration (MAC) for each species. Source: Steffey, 2009. Bronchospasm is associated with some diseases and other patient conditions and contributes to increased airway resistance. Among the earlier available volatile anesthetics, halothane was the most effective bronchodilator (Klide and Aviado, 1967; Coon and Kampine, 1975). Subsequent work with isoflurane, sevoflurane, and desflurane indicates the relaxation of constricted bronchial muscles by these agents is equal to or exceeds that caused by halothane (Hirshman and Bergman, 1978; Mazzeo et al., 1996; Rooke et al., 1997; Habre et al., 2001; Dikmen et al., 2003), although very deep anesthetic planes (≥2 times MAC) of desflurane may be associated with loss of bronchodilation, possibly due to release of tachykinin (Dikmen et al., 2003; Nyktari et al., 2006; Satoh et al., 2009). Volatile inhalation anesthetics may inhibit reflex hypoxic pulmonary vasoconstriction and thereby contribute to a maldistribution of ventilation to perfusion and an increase in the alveolar-to-arterial partial pressure of oxygen and decrease in PaO2 (Marshall et al., 1984; Miller et al., 2014). However, results of in vivo studies of clinically relevant concentrations of volatile anesthetics suggest minimal or no inhibitory effects (Mathers et al., 1977; Fargas-Babjak and Forrest, 1979; Domino et al., 1986; Lesitsky et al., 1998; Kerbaul et al., 2000, 2001). Airway irritation caused by inspiring an inhalation anesthetic is undesirable. Airway irritation has not been generally recognized as a problem associated with induction of anesthesia in animals. However, human patients commonly react by breath holding, coughing, and laryngospasm when exposed to concentrations of 7% or more desflurane early in the course of anesthesia (Eger, 1993; TerRiet et al., 2000). As a result, desflurane is not commonly used for anesthetic induction in human patients. Inhalation anesthetics may produce drug specific and/or dose-related effect. Such effects may manifest as changes in arterial blood pressure, cardiac output, stroke volume, heart rate, and/or rhythm of heart beat. Such changes may be caused by effects on myocardial contractility, peripheral vascular smooth muscle, and/or autonomic nervous system tone. Effects imposed by inhalation anesthetics may be further impacted by controlled versus spontaneous ventilation, concurrently administered drugs with direct or indirect hemodynamic actions, and preexisting cardiovascular disease. All of the volatile anesthetics decrease cardiac output, usually via a decrease in myocardial contractility (Eger, 1981; Eisele, 1985; Pagel et al., 1991b; Boban et al., 1992; Warltier and Pagel, 1992; Pagel et al., 1993) and, in turn, stroke volume. The magnitude of change is dose related and dependent on the agent (Klide, 1976; Steffey and Howland, 1978a, 1980; Eger, 1981; Merin et al., 1991; Warltier and Pagel, 1992; Mutoh et al., 1997; Hanouz et al., 2000). Arterial blood pressure is also decreased in a dose-related manner (Figure 11.8) (Steffey et al., 1974b, 1974c; Steffey and Howland, 1977; Merin et al., 1991; Frink et al., 1992b; Mutoh et al., 1997). The decrease in arterial blood pressure is usually related to a decrease in cardiac output and a decrease in peripheral vascular resistance, the relative contributions of which varies between species. Figure 11.8 Inhalation anesthetics dose-dependently (expressed as multiples of MAC) decrease mean arterial blood pressure (MAP) in mechanically-ventilated normocapnic dogs. Source: Data from Steffey et al., 1975; Steffey and Howland, 1978a; Steffey et al., 1984; Merin et al., 1991; Frink et al., 1992b. All volatile anesthetics depress myocardial contractility via alterations of intracellular calcium homeostasis at several subcellular locations in normal cardiac muscle (Bosnjak et al., 1991; Davies et al., 2000; Huneke et al., 2001; Park et al., 2007). The rank order of depression of contractile function is halothane = enflurane > isoflurane = desflurane = sevoflurane (Miller et al., 2014). Volatile anesthetics can provide important beneficial effects on mechanical function during myocardial ischemia and myocardial reperfusion injury. For a more complete review of mechanisms of action of volatile anesthetics on myocardial function readers are referred to the recent review by Pagel and colleagues (Miller et al., 2014). Inhalation anesthesia may alter the normal distribution of blood flow to organs. Specific effects are agent, dose, and time related (Vatner and Smith, 1974; Manohar and Parks, 1984a, 1984b; Manohar and Goetz, 1985; Bernard et al., 1991; Merin et al., 1991; Hartman et al., 1992; Eger et al., 2003a). For example, while volatile anesthetics cause a dose-dependent increase in cerebral blood flow, similar circumstances may decrease blood flow to the liver and kidneys (Gelman et al., 1984a, 1984b; Miller et al., 2014). Temporal changes in cardiovascular function have also been reported in a variety of animals during inhalation anesthesia (Vatner and Smith, 1974; Dunlop et al., 1987; Steffey et al., 1987a, 1987b, 1987c, 1987d). Inhalation anesthetics may increase the automaticity of the myocardium (Price, 1966). This effect is exaggerated by adrenergic agonists (Katz and Epstein, 1968). N-alkane anesthetics such as halothane sensitize the heart to arrhythmogenic effects of catecholamines (Raventos, 1956; Joas and Stevens, 1971; Munson and Tucker, 1975; Johnston et al., 1976; Eger, 1981; Weiskopf et al., 1989; Moore et al., 1993; Navarro et al., 1994; Miller et al., 2014); this side effect is not apparent with conventional ether agents. Hepatocellular injury may result as a general consequence of a reduction in hepatic blood flow or by direct volatile anesthetic agent toxicity. The chlorinated methanes, including chloroform, remain a classic research model for induction of hepatic injury (Plaa, 2000). However, of the contemporary volatile agents, liver injury is occasionally reported with halothane (Pohl et al., 1989; Kenna and Jones, 1995). Newer ether-type anesthetics have much less pronounced effects on hepatic blood flow (Merin et al., 1991; Bernard et al., 1992; Crawford et al., 1992; Frink et al., 1992b; Hartman et al., 1992), and isoflurane and desflurane undergo comparatively trivial biotransformation (Holaday et al., 1975; Ghantous et al., 1991; Njoku et al., 1997; Eger et al., 2002). Although inhaled agents are commonly associated with postanesthetic nausea and vomiting in people, emesis in the recovery period appears uncommon in most domestic animals.
Inhalation Anesthetics
Volatile
Gas
Major use
Isoflurane
Nitrous oxide (N2O)
Desflurane
Sevoflurane
Minor use
Halothane
Xenon (Xe)
Enflurane
Methoxyflurane
Diethyl ether
Physiochemical Characteristics
Chemical Characteristics
Physical Characteristics
Gas versus Vapor
Property
Desflurane
Halothane
Isoflurane
Sevoflurane
Enflurane
Methoxyflurane
Molecular weight
168
197
185
200
185
165
Liquid specific gravity (20°C) (g/ml)
1.47
1.86
1.49
1.52
1.52
1.42
Boiling point(°C)
23.5
50
49
59
57
105
Vapor pressure
20°C (68°F)
664
244
240
160
172
23
24°C (75°F)
798
288
286
188
207
28
ml of vapor/mL of liquid at 20°C
209.7
227
195
183
198
207
Partition coefficients
saline/gas (37°C)
0.287
0.825
0.517
0.329
0.713
4.0
olive oil/gas (37°C)
19.2
224
98.9
51.3
103
611
Methods of Description
Vapor Pressure
Properties Influencing Drug Kinetics: Solubility
Agent
Blood/gas
Oil/gas
Brain/blood
Heart/blood
Liver/blood
Kidney/blood
Muscle/blood
Fat/blood
Desflurane
0.45
19
1.2
1.2
1.5
0.9
1.7
29
N2O
0.46
1.4
1.1
1.0
–
–
1.2
2.4
Sevoflurane
0.65
47
1.7
1.7
2.0
1.2
2.6
52
Isoflurane
1.4
98
1.6
1.6
1.9
1.0
2.6
50
Enflurane
2.0
96
1.4
–
1.9
1.0
1.1
42
Halothane
2.4
224
1.9
1.7
2.3
1.3
2.9
57
Methoxyflurane
15
970
1.3
–
1.9
0.7
1.0
60
Xenon
0.12
1.8
1.6
–
–
0.9
0.9
–
Blood/Gas Partition Coefficient
Oil/Gas Partition Coefficient
Other Partition Coefficients
Latex
Silicone
Polyvinyl
Agent
Rubber
Rubber
chloride
Polyethylene
Desflurane
19
–
35
16
N2O
1.2
–
–
–
Sevoflurane
29
–
68
31
Isoflurane
49
67
110
2
Enflurane
74
–
120
2
Halothane
190
165
190
26
Methoxyflurane
630
–
–
118
Pharmacokinetics: Uptake and Elimination of Inhalation Anesthetics
Delivery to the Alveoli
Inspired Concentration
Alveolar Ventilation
Removal from Alveoli: Uptake by Blood
Solubility
Cardiac Output
Alveolar to Venous Anesthetic Partial Pressure Difference
Tissue groupa
Vessel rich
Muscle
Fat
Vessel poor
% of body mass
10
50
20
20
% of cardiac output
75
19
5
<1
Anesthetic Recovery
Anesthetic Dose: The Minimum Alveolar Concentration
Anesthetic
Cat
Dog
Horse
Human
Methoxyflurane
0.23
0.29
0.28
0.16
Halothane
1.04
0.87
0.88
0.74
Isoflurane
1.63
1.30
1.31
1.15
Enflurane
2.37
2.06
2.12
1.68
Sevoflurane
2.58
2.36
2.31
2.05
Desflurane
9.79
7.20
8.06
7.25
Xenon
–
119
–
71
Nitrous oxide
255
222
205
104
Pharmacodynamics: Actions and Toxicity of the Inhalation Anesthetics
Central Nervous System
Respiratory System
Cardiovascular System
Cardiac Rhythm and Catecholamines
Liver
Gastrointestinal
Kidneys
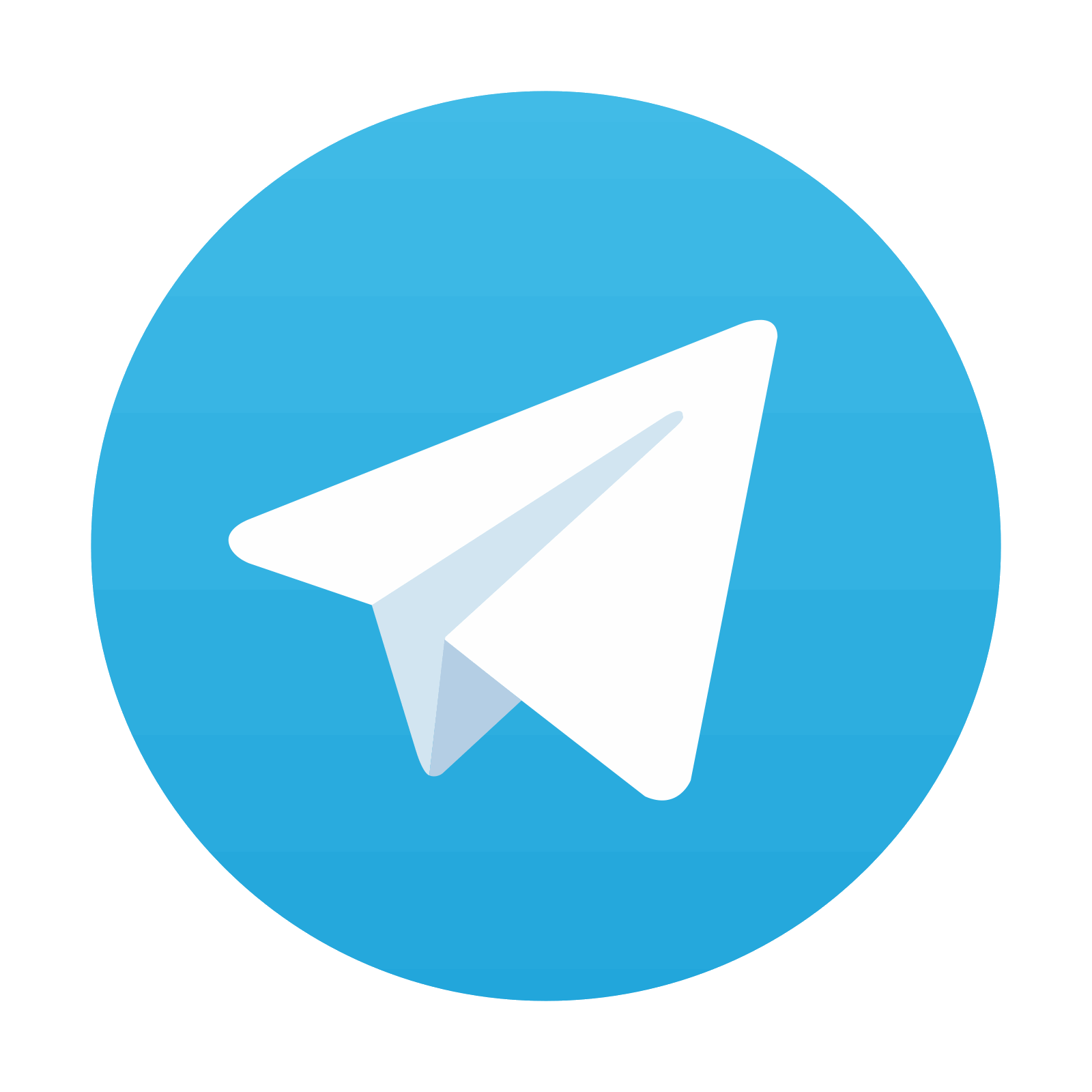
Stay updated, free articles. Join our Telegram channel
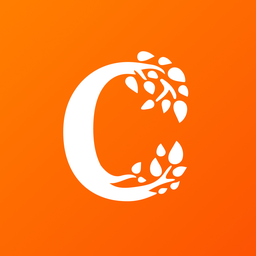
Full access? Get Clinical Tree
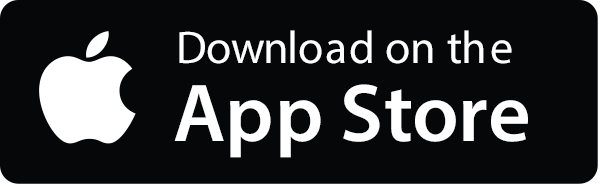
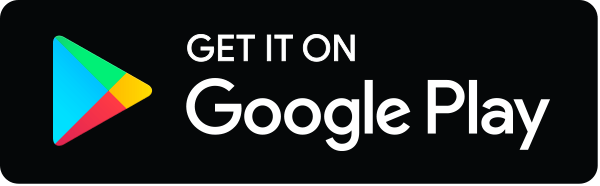