Chapter 7 Horses are cursorial animals and within the genus Equus different breeds have been developed for different occupations. Breeds specialized for racing tend to have long, gracile limbs, whereas draft breeds have shorter, stouter limbs in proportion to body size (Gunn, 1983). Fat and muscle distribution also differ between breeds but, regardless of breed, successful athletes tend to have body fat percentages in the range of 7.8–8.8% (Lawrence et al., 1992; Kearns et al., 2002). In athletic horses, muscles comprise about 53% of body mass compared with 42% in non-athletes, and the propulsive muscles in the hind limb account for a larger portion of that muscle mass (Gunn, 1987). These muscles are responsible for generating external work that is required for acceleration or to raise the center of mass when moving uphill or jumping (Clayton et al., 2002; Dutto et al., 2004a, b). The muscles in the proximal hind limb are characterized by having large physiological cross sectional areas (PCSA), associated with large mass and volume and relatively long fibers that move the joints through a large range of motion. Many of these muscles have multiple bellies: the more proximal bellies have both their origin and insertion located more proximally than the distal bellies allowing the entire muscle to exert force over a wide range of joint positions without needing long muscle fibers. The muscle fibers are arranged in series with elastic tissue and they attach to the bones directly or via short tendons. These characteristics are typical of muscles that are specialized for doing work. Many of the hind limb muscles are multi-articular and have complex fascial attachments and connections that make it difficult to separate extrinsic and intrinsic functions. Muscle volume and fiber length decrease, and pennation angles increase in a proximal to distal direction, so the muscles in the distal hind limb are smaller and less powerful with short, pennate fibers that are well-suited for generating force economically. Muscle size and fiber length are not correlated, however, so the larger muscles do not necessarily have longer fibers and smaller muscles do not always have shorter fibers (Table 7.1). Fiber length variability is low in the digital flexor muscles both within an individual horse and between different horses. The amount of force transmitted to the tendon is calculated as the product of PCSA and the cosine of the pennation angle. When pennation angle exceeds about 20° it significantly reduces the component of force transmitted to the tendon. The benefit of larger pennation angles is the increase in force-generating capacity as a result of the larger PCSA for a given volume. These muscular characteristics are typical of musculotendinous units used for elastic energy storage and recoil, which reduces the need for energetically expensive changes in muscle length, while minimizing distal limb mass. The suspensory ligament represents the end point of such modification, having minimal muscular tissue in the adult horse (Klomkleaw et al., 2002). Some muscles, notably fibularis tertius and superficial digital flexor, have so little muscular tissue that they function as strong tendinous bands to synchronize flexion–extension movements of stifle and tarsus in an arrangement known as the reciprocal apparatus (Wentink, 1978b). Table 7.1 Architectural properties of the muscles of the equine hind limb Data from Payne et al. (2005). During trotting, changes in potential and kinetic energy of the horse’s center of mass are in phase, which allows the distal limb to make substantial contributions to elastic energy storage (Biewener, 1998). In canter and gallop, the relationship between kinetic and potential energy varies during the stride (Minetti et al., 1999), which limits the ability to store elastic energy in the distal limb. It has been estimated that the hind limbs are responsible for two-thirds of the elastic energy savings in horses (Biewener, 1998). Payne et al. (2005) described the morphology and architecture of the hind limb musculature in horses of different sizes (body mass 430–600 kg) and ages (10–30 years) (Table 7.1). Data for muscle mass were scaled to body mass and measurements of fiber length were scaled to (body mass (kg) )1/3, which resulted in similar values among the seven subjects for most muscles, with the exceptions of gluteus medius and the hamstrings, which may have adapted in response to different types of training. After scaling for geometric similarity, fiber lengths varied more than muscle masses, particularly in the vertebral head of semitendinosus and in semimembranosus. In general, the hamstring group is capable of generating large forces and high powers. All the hind limb muscles have pennation angles greater than 20° and many have considerably larger angles. Most of the proximal muscles have little, if any, tendon and, when tendon tissue is present, it is light in weight (5.3–34.2 g) compared with tendons in the distal limb (44.8–208.7 g). Hind limb muscle architecture has been compared in Arabians, a breed that excels in endurance races, with Quarter Horses, a breed noted for its sprinting speed (Crook et al., 2008). Overall height and mass of the horses did not differ but the Quarter Horses had greater muscle mass and volume in both proximal and distal muscles and larger PCSAs allowing the development of larger forces (Crook et al., 2008). Thoroughbreds trained for sprinting have a higher hind limb muscle to body mass ratio than those trained for hurdling (Gunn, 1987). Fiber lengths and pennation angles are similar in different breeds of horses (Crook et al., 2008). This is in contrast to human sprinters who tend to have longer fibers and smaller pennation angles in their calf muscles compared with distance runners (Abe et al., 2000), though this may be an effect of a specific type of training. PCSA and calculated maximal isometric force decrease as follows: biceps femoris; semitendinosus; gastrocnemius; vastus lateralis; extensor digitorum longus; tibialis cranialis. Gluteus superficialis (Fig. 7.1, Table 7.1), the smallest of the gluteal muscles, is a hip flexor. It is one of the few muscles in the proximal hind limb that has a distinct tendon. This tendon is short in length (980 mm), small in mass (14.0 g) and volume (12.5 cm3) but has a relatively large cross-sectional area (1.39 cm2). It may provide some elastic energy storage and return. Calculated stress in the tendon is quite low (13.0 MPa). Gluteus medius (Fig. 7.1, Table 7.1) is a monoarticular hip extensor and is the largest muscle of the hind limb in terms of mass (8577 g) and PCSA (398 cm2). The fibers are long with pennation angles in the range 15–45° (Payne et al., 2005). These characteristics are indicative of a primary role in force and power generation. In fact, gluteus medius has been estimated to have by far the largest force-generating capacity of all the hind limb muscles and plays an important role in propulsion. Electromyographic studies at trot indicate that it is active during hind limb retraction in late swing and early stance (Robert et al., 1998). It contributes to a burst of power generation across the hip joint that pushes the trunk forward over the grounded hind limb (Clayton et al., 2001). The muscle fibers attach onto broad aponeurotic sheets that are somewhat compliant and may provide some elastic energy storage and return. Gluteus medius represents 2.0% of body mass in Quarter Horses and 1.7% in Arabians (Crook et al., 2008), which is indicative of the specialization of Quarter Horses for acceleration and speed. Internally, gluteus medius shows functional compartmentalization; the superficial parts of the muscle are composed primarily of type IIb fibers, which suggests a propulsive function, whereas the deeper parts have more type I fibers that are typical of a postural function (Lopez-Rivero et al., 1992; Serrano et al., 1996). Muscle fiber lengths do not appear to have a superficial to deep gradation and range from 135 mm to 300 mm throughout the muscle. Gluteus profundus (Table 7.1) is a smaller muscle than gluteus medius in terms of size and PCSA, with shorter fibers but similar pennation angles. Biceps femoris (Fig. 7.1, Table 7.1) is a multi-articular muscle that can act as a hip extensor, stifle flexor and tarsal extensor. It plays an important role in stabilization of the hip and stifle joints. The three heads (intermediate, vertebral and caudal) have a combined mass of 7928 g making it one of the largest muscles in the hind quarters. It has a large PCSA, especially the biarticular vertebral head, due to its large volume (Payne et al., 2005). This study reported pennation angles within the range of 20–55° in all three heads, whereas Crook et al. (2008) described a parallel fiber orientation (pennation angle, ≤5°) in the intermediate and caudal heads and 55° pennation angles in the vertebral head. Biceps femoris is the only muscle in the proximal hind limb that has a large tendon (mass, 106 g; CSA, 9.54 cm2) though this tendon is short in length (100 mm). During trotting, it is active during hind limb retraction in late swing and early stance (Robert et al., 1998). Semitendinosus (Fig. 7.1) has vertebral and pelvic heads. According to Payne et al. (2005), both heads have pennation angles in the range of 20–45° (Table 7.1) whereas Crook et al. (2008) identified distinct differences between the two head with the pelvic head having parallel fibers (pennation angle, ≤5°) and fibers in the vertebral head having a mean pennation angle of 23°, which supports its presumptive role in propulsion This muscle is thought to be important in generating large forces during hind limb retraction. PCSA of semitendinosus in Quarter Horses is double that of Arabians (Crook et al., 2008). Semitendinosus is active during retraction of both the ipsilateral and the contralateral hind limb in late swing and early stance (Robert et al., 1998). Semimembranosus (Fig. 7.1) has vertebral and pelvic heads that cannot easily be separated and are considered together in Table 7.1. A notable feature of this muscle is its large range of fiber lengths from 80–760 mm. Some fibers run the entire length of the belly from origin to insertion; others are staggered along the muscle belly. Similar to the other hamstring muscles, semimembranosus is multiarticular and is estimated to have the capacity to develop large force and high power (Payne et al., 2005). Adductor has two parts (magnus and brevis) that are united, and so are considered together in Table 7.1. Like semimembranosus, the adductor is a large muscle characterized by a wide range of fascicle lengths (80–390 mm) and it is estimated to produce large force and high power. Tensor fascia latae is estimated to be capable of producing moderate force and power (Fig. 7.1, Table 7.1). The tendon is relatively long (227 mm) but with a small CSA (1.35 cm2). Electromyographic studies indicate that this muscle is active during late swing and early stance (Robert et al., 1998). It is thought to play a role in stabilizing the stifle during the stance phase (Tokuriki & Aoki, 1995). Tibialis cranialis is a small muscle with a wide range of fiber lengths (17–218 mm) and highly pennate fibers (41°) capable of generating fairly high force but low power (Table 7.1). It is notable for having a short (92 mm), thick (CSA, 2.61 cm2) tendon of attachment (Payne et al., 2005). Fibers in the proximal part of this muscle are longer than those in the distal part (Crook et al., 2008) and it is the only muscle in the distal hind limb that has long fibers. Gastrocnemius (Fig. 7.1, Table 7.1) is the largest muscle of the distal limb. The medial and lateral heads combined have a volume of 1543 cm3 and mass 1625 g (Payne et al., 2005), with the lateral head being larger than the medial head but having shorter fiber lengths (Crook et al., 2008). The muscle belly contains extensive tendinous bands organized in series and in parallel and united by short (48–56 mm), pennate muscle fibers (see Table 7.3) (Payne et al., 2005). This architecture is consistent with a capacity for economical force generation via tendinous stretch and recoil within the muscle belly. As a consequence of the fiber pennation, the lateral head has the largest PCSA of all the hind limb muscles (644 cm2). The estimated force-generating capacity of gastrocnemius is quite high (8930 N) and is combined with a relatively large power-generating ability (244 W). The two heads have a thick (3.32 cm2) common (calcanean) tendon. Stress in the calcanean tendon has been estimated as 47 MPa (Ker et al., 1988), 30 MPa (Biewener et al., 1998) and 27 MPa (Payne et al., 2005). The gastrocnemius and cranial-tibial muscles may also play a role in centering the line of action of the resultant load on the tibia thus reducing the strain due to bending (Wentink, 1978a). Fibularis (peroneus) tertius forms the cranial arm of the reciprocal apparatus and is entirely tendinous (mass, 64.3 g; volume, 57.4 cm3; CSA, 1.59 cm2). Rupture of fibularis tertius as a consequence of hyperextension of the tarsus, does not have much effect on the horse’s stance or walking ability (Strubelt, 1928; Schamhardt et al., 1985), although Wentink (1978b) recorded slight hyperextension at the end of stance and less flexion of the tarsal joint during swing. At trot, the loss of elastic rebound of fibularis tertius is responsible for delayed protraction of the distal limb in early swing. Flexor digitorum superficialis (SDF) (Fig. 7.1, Table 7.1) in the hind limb is almost entirely tendinous, forming the caudal arm of the reciprocal apparatus that synchronizes stifle and tarsal motion in the sagittal plane. The muscle belly has a small volume of only 105 cm3 with uniformly short (1–6 mm) and highly pennate (40–60°) fibers, resulting in a surprisingly large PCSA (417 cm2) (Table 7.1). Estimates suggest that SDF has a capacity to generate high force (12500 N) but low power (17 W). The SDF tendon is long (748 mm) with a large mass (2.25 cm2) (Payne et al., 2005). As a consequence of having short muscle fibers and a long tendon, the hind limb SDF is particularly well suited to elastic energy storage and release and is more effective in this regard than the forelimb SDF (Brown et al., 2003). Flexor digitorum profundus (DDF) has three parts. Flexor digitorum medialis and tibialis caudalis are characterized by small volumes and short to medium length fibers (Fig. 7.1, Table 7.1), whereas flexor digitorum lateralis has a larger volume and short fibers (3–55 mm) embedded within large amounts of aponeurotic tendinous tissue that give the muscle belly a striated appearance on gross morphological examination (Payne et al., 2005). This muscle is estimated to have the capacity to develop high force (21100 N) but relatively low power (157 W) (see Table 7.3). Although the short muscle fibers and large PCSA are not compatible with active shortening of the musculotendinous unit, this architecture is well suited to work isometrically during elastic energy storage and release. Flexor digitorum lateralis is particularly effective in this regard, and more so than the humeral, radial or ulnar head of DDF in the forelimb (Brown et al., 2003; Payne et al., 2005). The DDF tendon has the largest cross-sectional area of the distal limb tendons (3.64 cm2). Stress in the flexor digitorum lateralis tendon has been estimated to be as high as 105 MPa (Ker et al., 1988) or as low as 40–50 MPa (Biewener et al., 1998). Tendons fail at stresses of approximately 120 MPa (Zajac, 1989), indicating that flexor digitorum lateralis may be operating close to, and may even exceed, its limit during isometric muscular contractions that generate high forces. In spite of this prediction, digital flexor tendon injuries do not occur frequently in the equine hind limbs. Extensor digitorum longus is a small muscle that generates a moderate force but little power (Fig. 7.1, Table 7.1). Its long (472 mm) tendon has a small cross-sectional area (1.13 cm2) and experiences low stress. Estimates of peak stress range from 14.4 MPa (Payne et al., 2005) to 36 MPa (Ker et al., 1988). In the standing horse, the body weight acts through the hip joint and is counteracted by the ground reaction force acting through the hoof. The net effect is to exert a compressive force on the hind limb. The joints are maintained in extension by the action of the extensor musculature. In general, the magnitude of extensor muscle force required to maintain the standing posture increases with body mass and with joint angulation. Thus, smaller animals tend to adopt a crouched posture with more flexion of the joints, whereas larger animals stand with more upright limb angulations to reduce loading of the extensor musculature (Biewener, 1989). Horses spend a considerable proportion of their time standing, which is facilitated by the presence of the passive stay apparatus that uses tendoligamentous structures to reduce the muscular activity required to maintain the standing posture (Dyce et al., 1996). The reciprocal apparatus is responsible for passively stabilizing the hind limb during standing. Extension of the stifle joint, which is the key to hind limb stabilization, is achieved by hooking the patella over the medial femoral trochlea (Sack, 1989). When the trochlea protrudes between the middle and medial parts of the patellar ligament, it holds the stifle in extension by mimicking the action of quadriceps femoris. Tension in the largely tendinous SDF prevents tarsal flexion and the more distal joints are stabilized passively by a system of ligaments and tendons. The equine patella is enveloped by the parapatellar fibrocartilage, which gives attachment to all parts of quadriceps as well as to tensor fascia latae, biceps femoris, gracilis and sartorius. During quiet standing with the patellar locking mechanism engaged, the only muscular force needed to stabilize the stifle is provided by tonic, low-level activity in vastus medialis, which inserts on the medial aspect of the parapatellar fibrocartilage. Tension in this muscle is estimated to be only 2% of the force that would be needed without the patellar locking mechanism (Schuurman et al., 2003). Upward fixation of the patella is an inability to disengage the patellar locking mechanism. It may be mild causing a slight hesitation in hind limb protraction or it can be severe enough to fix the entire hind limb in extension, effectively precluding locomotion. Contributing factors include shrinking of the patellar fat pad in underweight animals, abnormal coordination of muscles, such as vastus lateralis, that actively disengage the patella (Wentink, 1978a) or spastic activity in vastus medialis that precludes disengagement (Schuurman et al., 2003). Although kinematic gait analysis of the equine hind limb has been performed since the early part of this century (Walter, 1925; Krüger, 1938), the development of the computer gave new impetus to this field of research, both in the area of clinical applications (Fleiss et al., 1984; Kobluk et al., 1989; Martinez-del Campo et al., 1991; Back et al., 1995a, 1995b) and in computer simulation studies (van den Bogert & Schamhardt, 1993). The reciprocal apparatus, which couples stifle and tarsal joint motion, has been studied extensively (Strubelt, 1928; Molenaar, 1983; Wentink, 1978b; van Weeren et al., 1990). Back et al. (1995b) standardized the graphical presentation of kinematic data of the equine hind limb using joint angle–time diagrams in a large group of horses. The hip joint is the pivot point for rotation of the hind limb, and the ball and socket construction allows some motion outside of the sagittal plane. The more distal joints are constrained to move primarily in a sagittal plane with only small amounts of abduction–adduction and internal–external rotation (Lanovaz et al., 2002). Thus kinematic analysis in the two-dimensional sagittal plane captures most of the kinematic information describing hind limb movement patterns. Hind limb joint angles may be measured in several ways (Fig. 7.2): between the proximal and distal segments on the anatomical flexor aspect; as the angle by which the distal segment deviates from alignment with the proximal segment; or as some variation of these methods. The angle may be expressed in absolute terms or it may be normalized to the standing angle, the angle at ground contact or the average angle during the stride (Mullineaux et al., 2004). Three-dimensional kinematics of the tarsal joint have been measured using bone-fixed markers (Lanovaz et al., 2002) and will be described at the end of this chapter. When skin markers are used to represent sagittal plane motion of the hind limb segments, a minimum of two markers per segment are required. Typical marker configurations involve either placing a marker over the center of rotation of each joint or aligning two markers along the long axis of each segment (Fig. 7.3). Intra-limb coordination patterns can be visualized using stick figures or by joint angle–time graphs (Fredricson & Drevemo, 1972; Martinez-del Campo et al., 1991; Holmström et al., 1994; Back et al., 1994; Hodson et al., 2001, Dutto et al., 2006). Errors introduced into kinematic data due to skin movement relative to the underlying skeletal landmarks (van Weeren et al., 1992) may be small enough to be neglected on the crural and metatarsal segments, but are large enough to cause obvious changes in sagittal plane kinematics on the pelvic, thigh and pastern segments (Fig. 7.4). Errors as large as 15° in stifle angle and 30% in moment arm of gastrocnemius have been attributed to the effects of skin displacement in a walking pony (van den Bogert et al., 1990). Correction algorithms have been developed for many of the anatomical locations that are commonly used for marker placement for two-dimensional analysis of hind limb kinematics (van Weeren et al., 1992).
Hind limb function
Musculotendinous architecture
The stay apparatus
Sagittal plane analysis of hind limb kinematics and kinetics
Hind limb function
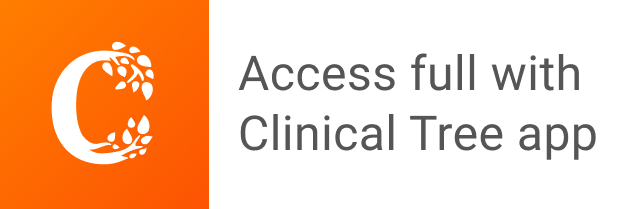