Carrie A. Schroeder Department of Surgical Sciences, School of Veterinary Medicine, University of Wisconsin, Madison, WI, 53706, USA The liver is a highly complex organ that is vital to several functions. Derangements in hepatic function can present many challenges to the anesthetist. Liver dysfunction can range from an elevation in liver enzymes with no clinical disease to fulminant liver failure. Fortunately, veterinary patients rarely present for anesthesia with severe liver disorders leading to hepatic failure such as cirrhosis or hepatitis. However, many liver conditions require surgical interventions such as liver biopsy or portosystemic venous shunt attenuation, and thus require sedation or general anesthesia. The anesthetist must understand the disease extent, the abnormalities associated with the disease, and their repercussions on drug metabolism and patient homeostasis before formulating an anesthetic plan. Blood flow to the liver comprises 25–30% of total cardiac output and is provided via dual blood supplies: the hepatic artery and portal vein. The portal vein accounts for 75% of the blood flow, but, owing to the relatively low oxygen saturation of portal venous blood, provides only 50–55% of hepatic oxygen requirements. However, the hepatic artery provides only 25% of blood flow, but 45–50% of oxygen requirements. Owing to the constitutively high hepatic blood flow, increases in hepatic oxygen demand are met with increased oxygen extraction from the blood rather than further increases in blood flow to the organ itself [1]. Under normal circumstances, decreases in cardiac output or systemic blood pressure with accompanying decreases in portal venous flow are usually compensated for by increases in hepatic arterial flow. The hepatic vasculature is richly innervated with alpha‐1‐adrenergic receptors; sympathetic nervous system activation results in hepatic artery vasoconstriction and, therefore, reduced hepatic blood flow [2]. Hepatic blood flow is also decreased in the face of increased portal pressure and is directly related to central venous pressure (CVP); such increases in CVP may be associated with congestive heart failure or positive pressure ventilation. Circumstances such as these will impair outflow of blood from the liver, increasing portal venous pressure and decreasing hepatic blood flow. In healthy animals, the liver provides multiple essential functions, which include bile formation and excretion, metabolic functions, plasma protein synthesis, and glycogen storage. Although the liver serves a wide array of functions, it has substantial reserve, and significant disease may be present before clinical abnormalities are evident. Bile salts and acids are synthesized in hepatocytes, and bile is carried from hepatic lobules by bile ducts, with the gallbladder serving as a reservoir in most species. The common bile duct releases bile into the duodenum on relaxation of the sphincter of Oddi. Morphine administration may cause spasm of the sphincter of Oddi in several species and was once contraindicated in the face of acute pancreatitis [3]. However, human research suggests that morphine is safe for analgesic use in acute pancreatitis and there is no clinical evidence to avoid the use of morphine in patients with hepatobiliary disease for this reason (see the section titled “Opioids”) [4]. Multiple enzymatic pathways are present in the liver, thereby metabolizing carbohydrates, proteins, fats, and pharmacological agents. Carbohydrate metabolism results in glucose synthesis and its storage in the form of glycogen. Abnormalities in glucogenesis can lead to hypoglycemia, a common presenting complaint in patients with severe hepatic dysfunction. Protein metabolism through deamination provides further vital energy substrates to the organism but produces ammonia as a by‐product. Under normal circumstances, ammonia is cleared from the body, but can be elevated in certain hepatic disease states and is a key contributor in the clinical presentation of hepatic encephalopathy (HE) [5]. Anesthetic and analgesic agent metabolism may also be impaired in the patient with hepatic disease, leading to prolonged drug effects. With few exceptions, most anesthetic agents undergo hepatic biotransformation to active or inactive compounds. Many of these reactions rely on the hepatic cytochrome P450 enzymes (CYP). The hepatic CYP enzymes can be induced by drugs such as barbiturates and inhibited by multiple drugs such as chloramphenicol, ketoconazole, and cimetidine (Table 4.1) [6–10]. Enzyme induction can cause a degree of drug tolerance and cross‐tolerance, thus requiring an increase in patient drug dosages, especially with chronic administration of enzyme inducers such as phenobarbital. Conversely, drug dosages should be decreased when enzyme inhibitors have been chronically administered, as enzyme inhibition may lead to a decrease in hepatic drug metabolism. Greyhounds appear to have decreased CYP activity, accounting in part for the increased anesthetic drug duration observed in this breed [8,10–12]. This has been extrapolated to all members of the sighthound family; drugs that rely heavily on the CYP system for biotransformation, such as methadone, should be titrated carefully in these breeds to avoid an exaggerated response [10]. Table 4.1 Enzyme inhibitors and inducers commonly encountered in veterinary medicine. Note that some pharmacological agents may act as both an enzyme inhibitor and an inducer, depending on the CYP enzyme form [6]. One of the most vital roles of the liver is protein synthesis. Nearly all proteins are formed in the liver. This includes albumin, coagulation factors, and cholinesterase [2]. Albumin is critical in providing plasma oncotic pressure and transport of multiple substances including anesthetic drugs. Hypoalbuminemia, azotemia, and acidosis reduce drug binding to albumin. This subsequently increases the free, unbound drug portion and potentially increases the drug effect. Albumin has a half‐life of approximately 21 days; due to this prolonged half‐life, acute liver disease typically will not manifest with hypoalbuminemia [1]. By contrast, all coagulation factors except III, IV, and VIII (thromboplastin, calcium, and von Willebrand factor) are synthesized by hepatocytes and, unlike albumin, have relatively short half‐lives; coagulation factors may be affected by acute liver disease [2, 6]. While liver dysfunction is often associated with prolonged coagulation times, various liver disease states may trigger a hypercoagulable state [13]. Patients with acute liver dysfunction should have coagulation testing performed before anesthesia and surgery. In addition to coagulations times such as prothrombin time (PT) and partial thromboplastin time (PTT), assays that evaluate the dynamic process of coagulation, such as thromboelastography (TEG), may be indicated if coagulopathy is suspected (see Chapter 14). Unfortunately, biochemical markers of hepatic dysfunction can be both nonspecific and insensitive (Table 4.2). Liver function tests include serum albumin, ammonia, bile acids, blood glucose, blood urea nitrogen (BUN), and cholesterol measurements [14]. Owing to variability in serum half‐lives, deviations in these markers of hepatic function may not be present immediately. Due to the relatively long half‐life of albumin (approximately 21 days), hypoalbuminemia may not be seen until hypoglycemia, hypocholesterolemia, and decreased BUN become evident. Coagulation factors have a relatively short half‐life, and as previously mentioned, abnormal coagulation may appear with acute disease. As abnormalities associated with liver disease may induce a hypocoagulable or hypercoagulable state, the patient’s overall coagulation status is best performed by TEG; however, many clinically relevant coagulopathies may be detected by evaluation of PT/PTT, platelet count, and fibrinogen measurement [15, 16]. Table 4.2 Biochemical markers and commonly encountered abnormalities associated with hepatic dysfunction. Hepatic enzyme elevations suggest hepatocellular damage rather than true dysfunction. The laboratory abnormalities associated with hepatocellular injury and evaluation of serum biomarkers of hepatocellular damage have been reviewed elsewhere [14]. In short, serum aminotransferases such as aspartate aminotransferase (AST) and alanine aminotransferase (ALT) are released with hepatocellular injury and are of some diagnostic use. AST is present in the liver as well as multiple extrahepatic sites, such as skeletal muscle and erythrocytes. ALT is found in large concentrations within hepatocytes and, as such, is more specific for hepatocellular damage that AST [14]. The absolute values of these enzymes can be related to the extent of hepatocellular injury but not the reversibility of injury; in general, injury that is less extensive is associated with a more minor increase in measured value as compared to the more significant enzyme elevations associated with widespread hepatocellular injury [14]. Serum alkaline phosphatase (ALP) is produced by the liver, bone, small intestine, and kidney. Mild elevations in this enzyme are consistent with hepatocellular injury, but elevations may also be present in pediatric patients, patients with bone disease, or patients chronically administered drugs such as phenobarbital, phenytoin, and corticosteroids [14]. Increased γ‐glutamyl transferase (GGT) is generally associated with cholestasis or biliary hyperplasia and is a more sensitive biomarker for hepatobiliary disease than ALP in cats [14]. A more sensitive and specific test for hepatobiliary dysfunction is preprandial and postprandial measurement of bile acids. Elevations in preprandial and postprandial bile acids can be present in portal venous shunts, cirrhosis, hepatic necrosis, and inflammation, steroid hepatopathy, cholestasis, and intestinal obstruction [17]. Ultimately, it is difficult to definitively diagnose hepatobiliary disease solely based on blood work. Diagnosis should be made by careful physical examination and clinical presentation in addition to biochemical evaluation. Diagnostic imaging, such as abdominal ultrasound or computed tomography (CT), and hepatic biopsy may be necessary to provide a definitive diagnosis. Of the modern anesthetics, there is limited direct adverse impact on hepatic function. However, it is important to note that nearly all injectable anesthetics and analgesics rely on varying degrees of hepatic biotransformation for drug elimination. While hepatic injury may not adversely impact the enzymatic processes of the liver, disease states that impact hepatic function such as portosystemic shunt (PSS) may result in altered drug pharmacokinetics and exaggerated or prolonged anesthetic effects. More common than true alterations in hepatic function are alterations in hepatic blood flow associated with general anesthesia. Decreases in cardiac output and mean arterial pressure by volatile anesthetics subsequently decrease portal blood flow in a dose‐dependent manner [2, 18]. Modern volatile anesthetics better preserve hepatic arterial blood flow, largely preserving total hepatic blood flow [18]; however, the overall impact of general anesthesia may result in altered drug pharmacokinetics administered in the perioperative period. Drugs with a low intrinsic clearance, with little reliance on the liver for metabolism, are minimally affected by changes in hepatic blood flow; drugs with a high intrinsic clearance are directly impacted by changes in hepatic blood flow [19]. Drugs with low intrinsic clearance relative to total hepatic blood flow, such as theophylline, will likely not have alterations in pharmacokinetics, while drugs with high intrinsic clearance relative to hepatic blood flow, such as morphine and ketamine, may have exaggerated or prolonged effects in the perioperative period. A number of other factors, including extrahepatic clearance of drugs, protein binding, and autoregulation of local blood flow, can profoundly affect anesthetic agent biotransformation and it may be difficult to predict the true clinical effects that may result from anesthetic‐induced alterations in hepatic blood flow. As is the case in when approaching an anesthetic plan in nearly all patients with coexisting diseases; an individualized plan, the use of agents with drug antagonists, and careful anesthetic titration are key to successful management in the perioperative period. Mechanical or assisted ventilation using high peak inspiratory pressures, mean pressures, and/or positive end‐expiratory pressures (PEEP) reduce hepatic blood flow because of venous congestion and decreases in both cardiac output and portal blood flow [20, 21]. On the other hand, hypercarbia, which can be associated with spontaneous ventilation under general anesthesia, can also decrease hepatic blood flow [22]. Since the liver and diaphragm/thoracic cavity are in close proximity, surgical manipulation may impinge on lung and thoracic wall excursions, resulting in hypoventilation. Care must be taken to reduce the mechanical restriction of breathing, especially in small patients. Mechanical ventilation may therefore be indicated in these cases. However, close peak airway pressure monitoring is necessary to avoid barotrauma and to avoid unnecessarily high airway pressures. Not only can perfusion of the liver and other organs be compromised by factors associated with general anesthesia, but the stress associated with surgery as well as mechanical factors can greatly impact hepatic blood flow. The hepatic vasculature is richly innervated with alpha‐1‐adrenergic receptors; activation of the sympathetic nervous system associated with surgical stress and pain may lead to catecholamine release resulting in an initial vasoconstriction, thereby reducing hepatic arterial flow [2]. Intra‐abdominal surgery can result in decreased hepatic blood flow due to the direct compression of hepatic vasculature by surgical manipulation; direct compression of vasculature leading to the liver either by manipulation of large tumors or by surgical instruments or hands may temporarily decrease arterial supply, portal blood flow, or venous return. Abdominal insufflation, utilized with laparoscopic techniques, may induce temporary increases in intra‐abdominal pressure (IAP) that may compromise splanchnic and abdominal organ blood flow. Intra‐abdominal hypertension (IAH) is defined as a sustained elevation in IAP above 12 mmHg, while pressures greater than 20 mmHg define abdominal compartment syndrome [23]. Most laparoscopic surgeries do not result in sustained pressures that may compromise blood flow, but other perioperative conditions such as fluid or blood accumulation, bowel distension, or abdominal wall rigidity may result in sustained IAH. Unfortunately, noninvasive means of measuring IAP are not readily available, but it is important to note that consequences of surgical interventions on hepatic blood flow may reach into the postoperative period and result in increased morbidity [23, 24]. Acepromazine is commonly used in veterinary medicine for its sedative properties. Acepromazine is a phenothiazine derivative that causes dopaminergic and alpha‐1‐adrenergic receptor blockade, leading to vasodilation [25]. Drug metabolism is via hepatic biotransformation, and no antagonist is available. As such, caution should be exercised when administering this agent to patients with hepatobiliary disease. The direct effects of acepromazine on hepatic blood flow and hepatic function have not been well characterized in veterinary medicine. The combined administration of ketamine, xylazine, and acepromazine does not appear to have adverse effects on liver function, graft liver regeneration, inflammatory response, or hepatic pathologic changes in rodent hepatic transplantation studies [26]. However, acepromazine should be used with caution in patients with hepatic disease; the vasodilation associated with acepromazine administration may lead to systemic hypotension [25]. Patients with hypoalbuminemia and/or patients presenting with PSS may have preexisting hypotension due to a decrease in colloidal osmotic pressure; acepromazine administration may further decrease systemic blood pressure. Furthermore, the coadministration of acepromazine and atropine induces abnormalities in platelet aggregation [27]. However, subsequent research evaluating the platelet function following acepromazine administration by TEG failed to detect platelet function inhibition in normal dogs [28]. Since patients with hepatic dysfunction may present with coagulopathies, caution should be exercised with acepromazine administration in the face of coagulation disorders or before procedures such as ultrasound‐guided liver biopsy. Caution should also be exercised in acepromazine administration prior to laparoscopic procedures, as systemic vasodilation may lead to splenic engorgement, decreasing visibility, or limiting available access points in the abdomen. Alpha‐2‐adrenergic receptor agonists such as dexmedetomidine offer excellent sedative properties at the cost of significant cardiovascular depression. Alpha‐2‐adrenergic receptor agonists significantly decrease heart rate and cardiac output while increasing systemic vascular resistance [29–31]. Dogs administered dexmedetomidine under experimental conditions demonstrated a delay in contrast delivery to the liver, suggesting decreased blood flow that was not antagonized by MK‐467, a peripheral alpha‐2‐adrenergic antagonist [32]. However, most studies suggest that hepatic blood flow may be well‐preserved. For example, dogs undergoing thoracotomy did not show significant decreases in hepatic blood flow after dexmedetomidine administration, and hepatic blood flow was preserved in human patients in early stages of septic shock when a dexmedetomidine infusion was administered. [33]. In fact, there are liver‐protective and other organ‐protective effects associated with dexmedetomidine administration [34]. Dexmedetomidine metabolism relies on hepatic biotransformation, but alpha‐2‐adrenergic receptor antagonists such as atipamezole are available in the event of increased effect or duration of effects. Overall, dexmedetomidine does not appear to have negative effects on the liver and is not contraindicated in patients with liver disease. However, caution should be used, as this agent can induce extreme sedation and cardiovascular depression and has not been extensively evaluated in veterinary clinical patients with preexisting liver disease. Careful dosage titration to the desired clinical effect and close monitoring of sedated patients are advised. The benzodiazepines, such as midazolam and diazepam, exert their sedative effect via enhancement of endogenous inhibitory neurotransmitter enhancement, namely, gamma‐aminobutyric acid (GABA) [35]. These agents have minimal cardiovascular effects and are generally well tolerated in patients with many disease states [36]. However, fulminant liver failure has been associated with oral administration of diazepam in cats [37]. This adverse event has not been reported after oral administration of alprazolam or parenteral administration of diazepam or midazolam, and both are considered safe for administration in the perioperative period. Metabolism occurs through hepatic biotransformation and a GABA receptor antagonist, flumazenil, is available if sedation is prolonged or excessive. Benzodiazepine administration for preoperative sedation of most patients with liver dysfunction is an excellent choice. However, care must be exercised in patients with HE. Neurons from animals with HE have increased sensitivity to benzodiazepines and increased “GABAergic tone” due to the presence of endogenous benzodiazepines, and the administration of exogenous benzodiazepines can aggravate HE [38]. The benzodiazepine antagonist flumazenil temporarily alleviates the HE symptoms, although there is no evidence for its use in providing long‐term management [39, 40]. Owing to the effect of HE on the benzodiazepine sensitivity, diazepam and midazolam dosages should be closely considered and may need to be greatly decreased or avoided in animals demonstrating hepatic encephalopathic signs. Opioids are classified based on their effects on opioid receptors [41]. Mu‐opioid agonists exert a pharmacological effect via activation of the mu‐opioid receptor; these agents include, but are not limited to, morphine, hydromorphone, fentanyl, and methadone. Butorphanol, classified as an opioid agonist–antagonist, acts as an antagonist at the mu‐opioid receptor and as an agonist at the kappa‐opioid receptor. Buprenorphine is a partial agonist at the mu‐opioid receptor and, as such, exhibits a submaximal clinical response. In general, opioids are safe for use in patients with liver disease. Most opioids rely on hepatic biotransformation, and the duration of effect may be prolonged in cases of liver dysfunction [42]. Similar to benzodiazepines, patients with HE may demonstrate an increased sensitivity to opioids, owing to increased opioid receptor density in the brain [43]. In patients with significant liver function compromise, drug dosages may need to be decreased and carefully titrated to effect. Naloxone, a nonselective opioid receptor antagonist, may be given if drug duration is prolonged or opioid agonist effects are profound. However, one must consider that most analgesic effects may also be reversed with the administration of naloxone. Locoregional anesthesia can supplement analgesic needs without inducing protracted sedation or systemic effects; for instance, the epidural administration of morphine successfully provides postoperative analgesic needs for patients undergoing surgical PSS attenuation [44]. As discussed previously, morphine contracts the sphincter of Oddi in several species, increasing biliary pressure [3]. Although previously considered unsafe for use in patients with acute pancreatitis, human research suggests that morphine is indeed safe for analgesic use in these cases [4]. At this time, there is no clinical evidence to suggest that morphine should be avoided in patients with hepatobiliary disease. Most opioids primarily undergo hepatic biotransformation followed by renal excretion. Remifentanil is a notable exception: metabolism is entirely extrahepatic, via ester hydrolysis [45]. It has an ultrashort duration of approximately 5 min and should be administered as a constant rate infusion to provide analgesia of any appreciable duration [46]. Remifentanil is advantageous in animals with liver disease, as duration of effect should be unchanged in the presence of liver dysfunction. In humans, duration and drug clearance were unchanged in severe, chronic liver disease and during the anhepatic phase of liver transplantation [47, 48]. Remifentanil has an isoflurane‐sparing effect in both dogs and cats and has been used in combination with isoflurane to provide anesthesia for liver biopsy in dogs [49–52]. As significant respiratory depression and bradycardia can result with bolus administration, close cardiovascular and ventilatory status monitoring is imperative, as anticholinergic administration and positive pressure ventilation may be required. Nonsteroidal anti‐inflammatory drugs (NSAIDs) are a critical component of most perioperative analgesic regimens and are administered chronically in many patients presenting for surgery and anesthesia. Using clinically relevant dosages, all NSAIDs have the potential to cause idiosyncratic hepatotoxicity with clinical signs of hepatic dysfunction and liver enzyme elevation, largely due to oxidative stress [53, 54]. Dogs treated with carprofen for postoperative pain exhibited acute ALT elevations without any clinical indicators of hepatobiliary dysfunction, while dogs chronically treated with carprofen did not demonstrate elevations in liver enzymes [55, 56]. In another study of long‐term administration of NSAIDs, meloxicam and etodolac were associated with increased GGT levels [57]. Overall, although not contraindicated, caution should be used when administering NSAIDs to animals with liver disease owing to the potential for this hepatotoxicity or oxidative stress. Furthermore, NSAIDs may adversely affect platelet aggregation. Platelet dysfunction occurred in dogs after administration of deracoxib and coagulation; coagulation times were increased in dogs receiving long‐term carprofen, meloxicam, ketoprofen, and flunixin [57–59
4
Hepatobiliary Disease
Introduction
Anatomy and Physiology
Enzyme inhibitors
Enzyme inducers
Ciprofloxacin
Rifampicin
Erythromycin
Phenytoin
Chloramphenicol
Phenobarbital
Ketoconazole
Omeprazole
Itraconazole
St. John’s wort
Fluconazole
Insulin
Fluoxetine
Dexamethasone
Omeprazole
Cimetidine
Ranitidine
Quinidine
Amiodarone
Chlorpromazine
Methadone
Propofol
Synthetic cannabinoids
Tramadol
Markers of Hepatic Dysfunction
Biochemical markers
Clinical signs and abnormalities
↑ Ammonia
Hepatic encephalopathy
↑ Bile acids
Ascites
↓ BUN
Hypotension
↓ Cholesterol
Impaired drug biotransformation
↓ Blood glucose
Coagulopathy
↓ Serum albumin
↑ Coagulation times (PT/PTT)
↓ K
↑ AST, ALT, ALP, GGT (nonspecific and often do not reflect true liver function)
Perioperative Effects on Hepatic Function
General Anesthetic Effects
Surgical Effects
Hepatic Dysfunction and Pharmacological Agents
Sedative Agents
Phenothiazines
Alpha‐2‐Adrenergic Receptor Agonists
Benzodiazepines
Opioids
Nonsteroidal Anti‐Inflammatory Agents
Stay updated, free articles. Join our Telegram channel
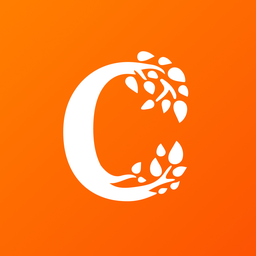
Full access? Get Clinical Tree
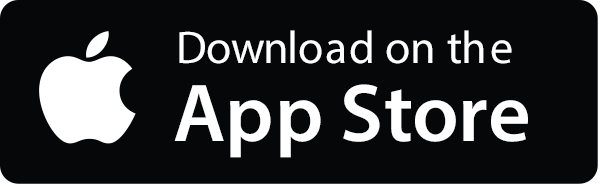
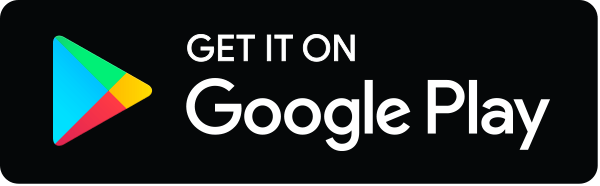