Hepatic, Pancreatic, and Metabolic Disorders
Basic Camelid Energy Metabolism
In addition to higher resting blood glucose concentrations, camelids clear exogenous glucose more slowly (Figure 41-1), have lower blood concentrations of fasting and stimulated insulin, and have considerably greater insulin resistance than cattle.1–6 The combination of high blood glucose and low blood insulin suggests a diabetes-like state. The source of the glucose is still being debated. Considering the unlikelihood of gastrointestinal (GI) absorption, exuberant hepatic gluconeogenesis appears the most likely source and fits with the low insulin environment. This is supported by evidence for a predominance of gluconeogenic enzymes over glycolytic enzymes in the camelid liver.7
Figure 41-1 Typical intravenous tolerance curves after rapid administration of 1 milligram per kilogram (mg/kg) of 50% dextrose solution in healthy adult llamas (blue) and alpacas (pink). Note that the peak glucose concentrations are lower in alpacas than in llamas and that neither species has returned to baseline by 4 hours.
Immunohistochemical examination has revealed what appear to be substantial populations of GLUT1, GLUT2, and GLUT3 in the appropriate places in New World camelids.8 GLUT4 was not found, but whether that was caused by the absence of the transporter or lack of cross-reactivity with the test antibody was not determined. GLUT4 was recently found by using the Western Blot test in camels, mainly in the diaphragm and masseter, and the authors suggested some deficiency in its migration to the cell surface.9 Insulin receptors have also been identified in camels and appear to be similar in form and function to those of other mammals.10
The relative lack of GLUT4 activity in camelids is most likely responsible for their partial insulin resistance. However, this insulin resistance may be reversed by using long-acting insulin or insulin continuous rate infusion (CRI), suggesting that adult camelids have the mechanism for a GLUT-4 response but that it is somewhat dormant due to chronic understimulation.11 This further suggests that underproduction of insulin is the primary disorder and peripheral resistance is secondary.
The blood insulin concentrations of fasted adult camelids are roughly one half to a third of those of a variety of other mammals.1,2 Interestingly, neonatal crias have blood insulin concentrations, glucose tolerance, and insulin sensitivity more in line with those other mammals, which suggests that a major change occurs during or after weaning.12 The most obvious changes are in the diet and forestomach digestion. Most dietary carbohydrate in adults is fermented to VFAs, and forestomach bypass ceases with the end of nursing. It is possible that camelids, with their longer gastric retention times and highly efficient fermentative digestion, prevent even more carbohydrate from reaching the intestine compared with ruminants. Carbohydrate in the intestines triggers incretin release, which potentiates the subsequent insulin response to hyperglycemia. The best known incretins are gastric inhibitory polypeptide (GIP, also known as the glucose-dependent insulinotropic peptide) and glucagon-like peptide 1 (GLP1), released by the duodenum and the ileum, respectively. Adult camelids treated with exogenous GLP1 have glucose tolerance and circulating insulin concentrations resembling those of crias or monogastrics, which suggests that lack of incretins is, indeed, the cause of camelids’ poor insulin production and therefore central to their exuberant gluconeogenesis, poor glucose tolerance, low insulin production, and partial insulin resistance.13 Further unpublished work supports this.
The consequences of impeded insulin production on glucose metabolism in the healthy camelid are several. Gluconeogenesis appears to occur unimpeded, increasing camelids’ needs for propionate or amino acid precursors. Stimulation for glycogen storage is less, possibly giving camelids smaller glycogen reserves compared with ruminants.14 Most importantly, or at least most dramatically on blood evaluation, camelids have little ability to counteract hyperglycemia, which leaves them prone to high and prolonged glucose peaks. These consequences may become even more significant in the sick camelid.
The feed-deprived camelid begins to mobilize NEFAs from peripheral adipose stores within 8 hours. As far as we know, this intracellular lipolysis occurs under similar hormonal conditions as in other species; that is, catecholamines and possibly other hormones stimulate lipolysis, whereas insulin inhibits it.14 Glucocorticoids appear to have little effect.15 Insulin inhibits lipolysis at lower concentrations than it controls hyperglycemia, so healthy camelids are able to regulate fat mobilization better than blood glucose concentrations but are perhaps more vulnerable to disease induced by slight reductions in insulin production compared with ruminants.
The free fatty acids (FFAs) liberated from adipose tissue circulate bound to albumin. The consequences of hypoalbuminemia, a common finding in sick camelids, on this transport are unknown. FFAs are taken up by cells in proportion to their blood concentrations and may be oxidized by most tissues for energy. However, metabolically active cells may not meet their energy needs through NEFAs alone.
Disorders of Carbohydrate Metabolism
Stress Hyperglycemia
Stress hyperglycemia is a well-known phenomenon in camelids. Ruminants and horses rarely develop hyperglycemia of greater than 300 mg/dL, and stressed camelids occasionally have blood glucose concentrations of 500 mg/dL (27.5 mmol/L) or more. Forty-two percent of camelid patients at our clinic have hyperglycemia on initial evaluation, with about 7% having greater than 300 mg/dL (16.5 mmol/L). The frequency and severity of stress hyperglycemia most likely relates to the poor mechanisms for glucose clearance and inability to counteract glucogenic stimuli. Sources of increased blood glucose include absorption from the diet (suckling animals only), administration of exogenous glucose, accelerated gluconeogenesis, and glycogenolysis. Epinephrine and cortisol both appear to mobilize glycogen stores in camelids, as in other species. Epinephrine leads to near-instantaneous increases in blood glucose.14 Cortisol takes longer (90 to 120 minutes) but leads to longer-lasting, higher peaks.15 Both hormones may also induce gluconeogenesis. Although the glycogen stores in camelids appear to be relatively small, epinephrine, cortisol, and other glucogenic factors are likely to increase blood glucose to a greater degree or for a longer duration in camelids than in other species because of the lack of effective countering by insulin.
Diabetes Mellitus
Diabetes mellitus has been described empirically but has been reported scientifically in only one camelid.16 That camelid was reported to have type I diabetes mellitus, even though it had a blood insulin concentration within reference ranges for camelids.
Hyperosmolar Disorder
Hyperosmolar disorder is one consequence of persistent or severe hyperglycemia, usually in conjunction with restricted fluid intake.17 This disorder is most severe in young crias, especially orphans or other bottle-fed crias, but occurs to some degree in camelids of all ages. Factors such as endogenous or exogenous catecholamines or glucocorticoids, pancreatic insufficiency, or exogenous glucose, which increase exogenous glucose, are poorly countered by endogenous insulin, allowing hyperglycemia to develop. In neonates, feeding cow or goat milk or a milk replacer instead of camelid milk may also increase the risk, as camelid milk appears to have an insulin-like effect.
Hyperadrenocorticism or Glucocorticoid Administration
Endogenous glucocorticoids are harder to assess. They have long been blamed for stress hyperglycemia, but because of their delayed onset of efficacy, they are likely only contributing to hyperglycemia in camelids stressed for 2 hours or more.15 Some abnormality of the pituitary–adrenal axis may be responsible for persistent hyperglycemia and diabetes-like signs in certain camelids, although this has not been investigated sufficiently.
Pancreatic Necrosis
Pancreatic necrosis with damage to islet cells could be another possible cause of hyperglycemia.18 Both acute and chronic forms have been reported but not any effects on glucose homeostasis. Acute pancreatic necrosis appears to cause colic signs, and both forms appear to affect peripancreatic and intrapancreatic fat more than they affect the pancreatic parenchymal cells.
Chronic pancreatic atrophy with islet reduction has also been described and possibly associated with a picorna virus similar to equine rhinovirus.19 Diabetes-like clinicopathologic changes and glucose responses have been described. However, recent work suggests that these assessments of pancreatic form and function were normal for camelids.
Hypoglycemia
Hypoglycemia is an uncommon finding in camelids, even in sick neonates and older camelids with longstanding anorexia. Poor glucose clearance limits its rate of use, even in camelids lacking other forms of energy, and exuberant gluconeogenesis continues in spite of negative energy balance.20,21 Hypoglycemia usually reflects abnormal consumption or impaired gluconeogenesis. Examples of the first are septic camelids or those with Mycoplasma haemolamae infection, in which hypoglycemia may develop from bacterial consumption. With these disorders, bacterial consumption continues in blood samples, so delayed laboratory analysis may yield misleading results with low levels, and the prevalence of hypoglycemia even in these populations is lower than expected. Iatrogenic hypoglycemia following insulin administration also is the result of increased consumption. This occurs most commonly in neonates or any camelid administered a long-acting form of insulin without simultaneous glucose administration. Lactating or pregnant camelids may also be at higher risk because of greater insulin-independent glucose uptake.
Hypoglycemia is best treated by intravenous glucose administration. A bolus of 0.25 grams per kilogram (g/kg) of dextrose should increase blood glucose concentration by 50 to 100 mg/dL (up to 5.5 mmol/L). Slow infusions are also effective. Because of the infrequency of this disorder and the potential for aggravating hyperglycemia, confirmation of hypoglycemia before treatment is advisable.
Monitoring Disorders of Carbohydrate Metabolism
Measurement of blood glucose is relatively straightforward and informative. Blood insulin may be measured by radioimmunoassay, but the standard curve must be adjusted for the very low insulin concentrations found in camelids.1 Glucose tolerance tests and insulin response curves may provide evidence about glucose clearance and insulin resistance, but, again, the slower clearance and lower insulin sensitivity of camelids must be kept in mind when interpreting results.1,5
Treatment of Disorders of Carbohydrate Metabolism
As glucose itself appears to be marginally useful to camelids and the chance of deficiency in low, most efforts are directed at avoiding the complications of hyperosmolality. Transient, severe hyperglycemia may be left to resolve on its own, as long as the camelid remains adequately hydrated. If glucose remains high or the rise in blood sodium concentration is becoming a concern, insulin may be administered to reduce blood glucose. Regular insulin (0.2 units/kg) given intravenously (IV) has a near-instant effect that lasts about 1 hour.5 Regular insulin also may be give as a constant rate infusion (0.02 units/kg/h [0.009 units/lb/hr]).22 Long-acting preparations given subcutaneously (0.4 units/kg) have effects lasting up to 24 hours.11 Newer forms such as insulin glargine appear to be more potent than older preparations and hence may be effective at lower doses (0.2 units/kg, q24h). Both porcine and human recombinant forms appear to be effective. Glucose should be monitored over the period of insulin efficacy to prevent hypoglycemia. Potassium supplementation may be necessary because insulin treatment tends to reduce extracellular potassium.
Treatment involves addressing hyperglycemia and fluid administration. Oral fluids are preferable to parenteral fluids, if the digestive system is functional, because the slower rate of absorption decreases the chance for rapid shifts in body water. Up to 3% of body weight may be administered orally or by tube at one time. This may be repeated up to three times a day. Free-choice oral water is also an option and rarely leads to complications. Milk or a diluted milk replacer may be used in lieu of water in suckling crias. If used, IV fluids should be administered at near-maintenance rates. Initial crystalloid fluid boluses of 2% to 5% of body weight may be tolerated and may restore renal output. Blood sodium should be measured periodically to avoid worsening of hypernatremia or too rapid correction. Decreasing blood sodium up to 0.5 mEq/L/hr appears to be safe. If hypernatremia is worsening, diluting isotonic salt solutions with 5% dextrose or adding low-sodium oral fluids to the treatment regimen may help. If correction is too rapid, signs of cerebral edema may appear. Administration of diluted fluids should stop until the edema is addressed and antiseizure medications may be necessary (see Chapter 38). Antibiotics, antiinflammatory medications, plasma, or other treatments may be indicated for the primary disease. Because of the role of steroids in stimulating hyperglycemia and retaining sodium, their use should be avoided in the treatment of hyperosmolar disorder.
Disorders of Lipid Metabolism
Exuberant Lipid Mobilization (Ketosis, Ketonemia, Acetonemia)
Exuberant lipid mobilization (ketosis, ketonemia, acetonemia) is a catch-all term referring to camelids with high NEFA or BOHB levels which have not developed hepatic lipidosis. It is generally believed that this state precedes and may progress to lipidosis.20 It is characterized by general malaise and inappetence, occasionally with weight loss and often with some more obvious disease. It is essentially a laboratory diagnosis, defined by abnormal blood NEFA or BOHB values. Approximately two thirds of all sick camelids have increases in one or both of these determinants, with increases in NEFA values being slightly more common than increases in BOHB values. This is separated from dietary insufficiency because a decrease in feed intake usually occurs and also because it is believed that the hormonal milieu (high catecholamine, low insulin) stimulates lipolysis and ketogenesis beyond that which is normal for fasted camelids. This disorder is treated by using the general strategy outlined below, and it is usually thought that treating at this stage may prevent the camelid from developing hepatic lipidosis.
Hepatic Lipidosis
Hepatic lipidosis appears to represent a progression of exuberant lipid mobilization or possibly hyperlipemia. Specifically, it refers to microscopic fat deposition within hepatocytes (Figure 41-2) although lipid accumulates in renal tubular cells and other tissues as well. Lipidosis is thought to be caused either by increased fat (NEFA) delivery to tissues, or by inhibition of hepatic fat oxidation or export. Unlike with related disorders in cattle or sheep, lipidosis is reasonably common even in nonpregnant, nonlactating camelids and has been seen in both genders and in crias as young as 2 days old.23 In ruminants, negative energy balance and carbohydrate insufficiency are thought to be important in the pathogenesis, but camelids with all fat mobilization syndromes have a high prevalence of hyperglycemia and often have no extraordinary energy demand. Therefore, it is presumed that some factor beyond dietary insufficiency contributes to the condition. In rare cases, lipidosis in newborns may be a physiologic result of their high-fat milk diet, but that is unlikely to be a factor in sick crias.
Figure 41-2 Microscopic appearance of moderate hepatic lipidosis. Note that the cells containing lipid vacuoles extend from near the portal triads (PT) to the central vein (CV), without the concentrations around the central vein often seen in cattle.
In addition to simple starvation or competition for food, various stressors may promote lipolysis. These include transport, extreme temperatures, hypoproteinemia, and illness. Concurrent or previous liver disease may compromise its function in energy metabolism. Hormonal mechanisms may also play a role, especially suppression of insulin production or increase in catecholamines. Epinephrine in particular has been shown to be a potent stimulator of adipose mobilization and antagonizes the actions of insulin as well.14
Hyperlipemia
Based on the Fredrickson classification of hyperlipemia, the disorder in camelids is most likely to be type IIb, with increases in both low-density lipoproteins (LDLs) and VLDLs. In humans, type IIB hyperlipemia may be familial or a component of metabolic syndrome (CHAOS [Coronary artery disease, Hypertension, Adult onset diabetes, Obesity, and Stroke] in Australia). It is associated with increasing age, genetic factors, sedentary lifestyle, and high caloric intake. In camelids, it usually arises in conjunction with another disorder such as endoparasitism, heat stress, hepatic lipidosis, CNS disorders, sepsis, or colic.24–27 As such, it is frequently viewed as a complication, usually near-terminal, of those disorders. Other factors associated with metabolic syndrome in humans include high blood pressure, central obesity, fasting hyperglycemia, insulin resistance, poor glucose tolerance, and possible microalbuminuria. Several of these characteristics are normal in camelids, and it may be that the preceding illness exacerbates the metabolic state by reducing blood proteins, by stimulating catecholamine release, or by decreasing or antagonizing insulin activity. As with lipidosis, all ages, signalments, and body conditions of camelid are affected by hyperlipemia.24
Few specific clinical signs are associated with hyperlipemia. As with other disorders of fat metabolism, anorexia, obtundation, weakness, progressing to recumbency and eventually neurologic signs and death are common findings. A small number of camelids with chronic hyperlipemia may develop lipogranulomatous ulcerative skin lesions, particularly of distal extremities. Hyperlipemia may also occasionally be recognized by cloudiness of the plasma or serum. In its milder form, this is difficult to recognize, but as the hyperlipemia worsens, the serum takes on a milky appearance. As a word of caution, hyperlipemia may falsely increase protein readings on the refractometer, causing hypoproteinemia to be missed.
Recent findings suggest mild hyperlipemia tends to worsen unless specifically treated, even if any underlying disorders are appropriately addressed.24 Thus, even mild hyperlipemia may represent the beginning of a serious, progressive metabolic derangement.
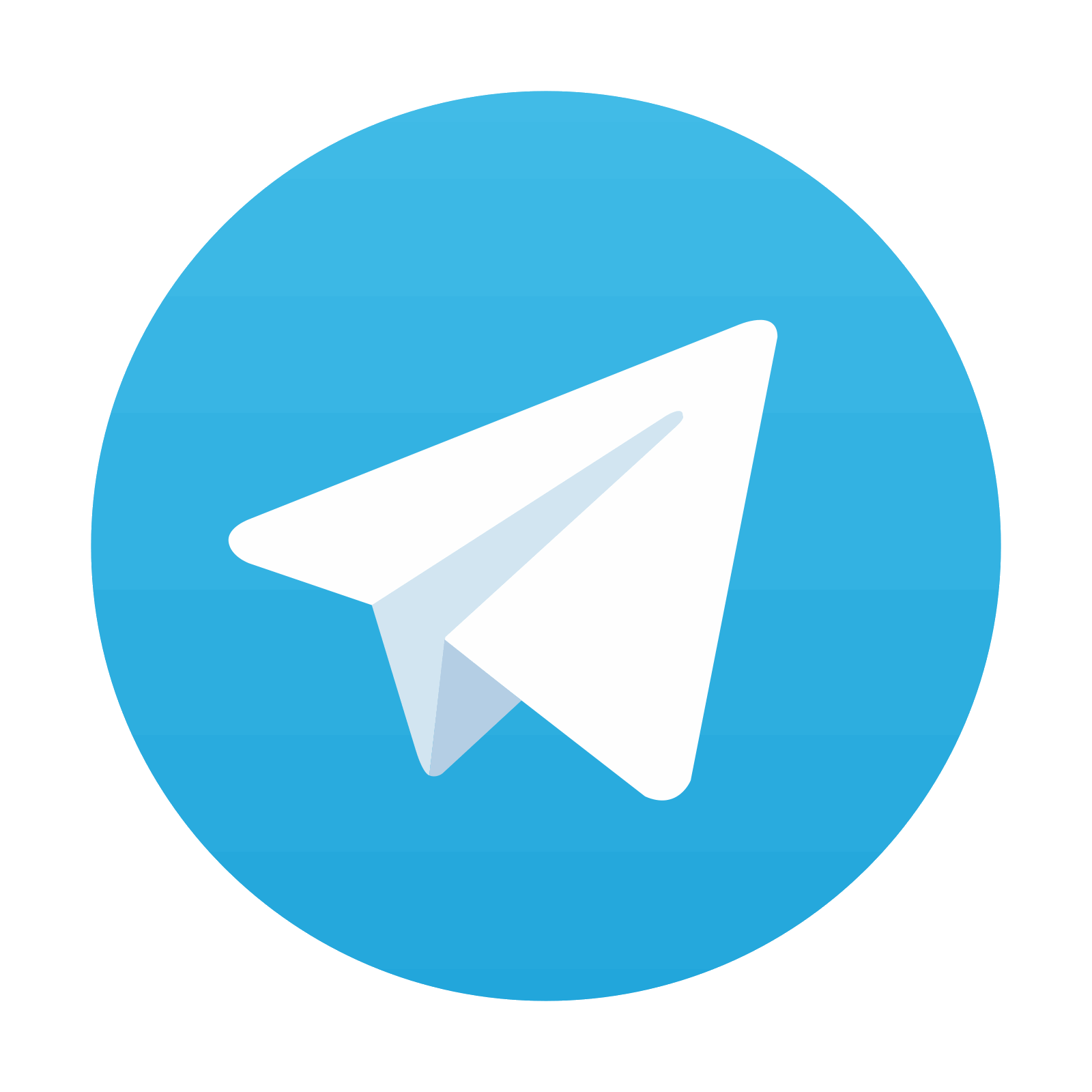
Stay updated, free articles. Join our Telegram channel
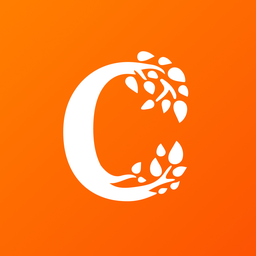
Full access? Get Clinical Tree
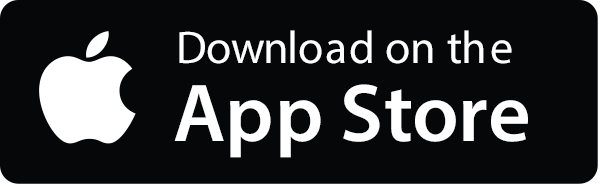
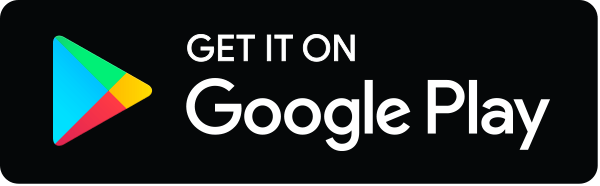