29 Duncan C. Ferguson and Margarethe Hoenig Glucocorticoids (GCs) are among the most widely used (and misused) class of drugs in veterinary medicine. In recent years, more has been learned about the actions and adverse effects in a variety of species but, nonetheless, the depth of scientific information on optimal glucocorticoid dosages and dosing intervals in many species is still quite variable as species differences in metabolism and action exist. Therefore, therapeutic protocols are often the product of clinical experience, common sense, and information from human medicine, or from another species. Though the following discussion emphasizes systemic use of glucocorticoids, it should be recognized that local application (ophthalmic, otic, intraarticular, topical, intralesional) also has similar systemic effects. Spontaneous glucocorticoid deficiency without concomitant mineralocorticoid deficiency is a relatively rare occurrence in dogs, the species that most commonly suffers from Addison’s disease (gluco- and mineralocorticoid deficiency). The underlying cause is suspected to be an immune-mediated destruction of the adrenal cortex, making replacement of the physiological hormones aldosterone and cortisol (in most domestic animals) to be the primary clinical goal. Spontaneous selective glucocorticoid deficiency is rare; however, exogenous glucocorticoids may themselves induce selective atrophy of the glucocorticoid-producing part of the adrenal cortex (zonae fasciculata and reticularis) (Addison, 1855; Ferguson, 1985a; Ferguson et al., 1978; Hoenig and Ferguson, 1991b). Glucocorticoids are potent antiinflammatory and immunosuppressant agents. The majority of therapeutic applications for these agents fall into these classifications. However, the adverse metabolic effects, as described in Section Toxicity, are difficult to separate pharmacologically from the therapeutic benefits, making glucocorticoids potent, yet potentially dangerous, compounds. The cellular basis for the action of these hormones has recently become clearer and has given rise to new pharmaceutical classes where the antiinflammatory/immunosuppressive and metabolic effects, for example, can be dissociated (see Section Newer Preparations) (Schäcke et al., 2007; Barnes, 2010; Hasselgren et al., 2010; Vandevyver et al., 2013). The adrenal cortex synthesizes a variety of steroids from cholesterol and releases them into the circulation. Those steroids with effects on intermediary metabolism are termed “glucocorticoids” and are produced mainly in the layers of the adrenal gland called the zonae fasciculata and reticularis. The steroids with primarily salt-retaining activity are called mineralocorticoids and are synthesized in the zona glomerulosa. The adrenal gland is also capable of synthesizing steroids with androgenic and estrogenic activity. The major glucocorticoid in most domestic animals is cortisol, and in most mammals, the most important mineralocorticoid is aldosterone. In some species (e.g., the rat), corticosterone is the major glucocorticoid. It is less firmly bound to protein and therefore metabolized more rapidly. Quantitatively, dehydroepiandrosterone (DHEA) is the major androgen, with part of it being sulfated to DHEA-sulfate. Both DHEA and androstenedione are very weak androgens. A small amount of testosterone is secreted by the adrenal gland and may be of greater importance as an androgen. Little is known about the estrogens secreted by the adrenal gland. However, the adrenal androgens such as testosterone and androstenedione can be converted to estrone in small amounts by nonendocrine tissues (Aron and Tyrrell, 1994; Tyrrell et al., 1994). The biochemical pathways involved in adrenal steroidogenesis are shown in Figure 29.1. The initial adrenocorticotropic hormone (ACTH)-dependent rate-limiting step is the transport of intracellular cholesterol from the outer to the inner mitochondrial membrane by steroidogenic acute regulatory (StAR) protein, where it is then converted to pregnenolone by cytochrome P450scc (SCC; side-chain cleavage enzyme). The synthesis of the various adrenal steroids then involves a series of cytochrome P450 enzymes (Table 29.1 ). SCC and the CYP11B enzymes are localized to the mitochondria and utilize a specific electron shuttle system to hydroxylate steroids. 17α-hydroxylase and 21-hydroxylase are localized in the endoplasmic reticulum. In the cytoplasm, pregnenolone is converted to progesterone by 3β-hydroxysteroid dehydrogenase (3β-HSD), which is the target of the antiadrenal drug trilostane. Progesterone is hydroxylated to 17- hydroxyprogesterone (OHP) by 17α-hydroxylase, and this step is necessary for glucocorticoid synthesis. 21-hydroxylation of either progesterone in the zona glomerulosa or 17-OHP in the zona fasciculata is accomplished by 21-hydroxylase, yielding deoxycorticosterone (DOC) or 11-deoxycortisol, respectively. Cortisol is then synthesized in the mitochondria by the conversion of 11-deoxycortisol to cortisol by the enzyme 11-β-hydroxylase. In the zona glomerulosa, 11β-hydroxylase also converts DOC to corticosterone. Aldosterone synthase also accomplishes this reaction and converts corticosterone to aldosterone through the intermediate 18-OH corticosterone. Figure 29.1 Adrenal steroidogenesis. Steroidogenic acute regulatory (StAR) protein mediates uptake of cholesterol into mitochondria within adrenocortical cells. Then aldosterone, cortisol, and adrenal androgens are synthesized through a series of steroidogenic enzymes. A’dione, androstenedione; DHEA, dehydroepiandrosterone; DOC, deoxycorticosterone. Source: Stewart, 2003. Reproduced with permission of Elsevier. Table 29.1 Nomenclature for adrenal steroidogenic enzymes and their genes. Source: Adapted from Stewart, 2003. Reproduced with permission of Elsevier. Adrenal steroids are metabolized in extradrenal tissues as well, and prereceptor enzyme-mediated metabolism of glucocorticoids adds another layer of metabolic control. Cortisol is interconverted with biologically inactive cortisone by the 11-β-hydroxysteroid dehydrogenase system (11-β-HSD). The conversion of inactive 11-ketoglucocorticoids cortisone and 11-dehydrocorticosterone into active 11-β-hydroxyglucocorticoids (cortisol and corticosterone, respectively) is catalyzed by 11-β -HSD1 using NADPH. This enzyme is prominent in metabolically active tissues such as the liver, skeletal muscle, and fat. Likewise, the synthetic glucocorticoid prednisone must be converted by 11-β-HSD1 in the liver for bioactivity (Stewart, 2003). Conversely, 11-β-HSD2 uses NAD+ to convert cortisol to cortisone, essentially inactivating it. In the distal renal tubules, this enzyme inactivates cortisol ensuring that only aldosterone is an agonist at the mineralocorticoid receptors (Atanasov and Odermatt, 2007; Divari et al., 2011; Chapman et al., 2013). The placenta and fetus also express 11-β-HSD2, thereby preventing premature developmental programming that might otherwise be caused by cortisol. Production of ACTH is stimulated by corticotropin-releasing hormone (CRH), which is a hypothalamic hormone (Figure 29.2) (see also Chapter 26); production of ACTH is also influenced by vasopressin (antidiuretic hormone; ADH), particularly during stress. There is also central nervous system (CNS) input into hypothalamic hormone secretion. A “short-loop” feedback system of ACTH on the corticotrophs (ACTH-producing cells) in the pituitary has also been described. In humans, cortisol is secreted in response to pulsatile ACTH release with diurnal variation. Figure 29.2 Hypothalamic–pituitary–adrenal axis. Exogenous glucocorticoids inhibit the hypothalamic and pituitary function in a negative-feedback fashion. ACTH, through “short-loop” feedback, inhibits its own production. Therefore, recovery of the entire hypothalamic–pituitary–adrenal axis requires withdrawal of glucocorticoid and is not enhanced by the administration of exogenous ACTH. CNS, central nervous system; CRH, corticotropin-releasing hormone; ACTH, adrenocorticotropic hormone; + represents stimulation; − represents inhibition. Negative feedback of glucocorticoids on ACTH secretion occurs at both the hypothalamic and pituitary levels via two mechanisms: Pharmacological doses of glucocorticoids have a profound effect on endogenous glucocorticoid regulation, suppressing both hypothalamic and pituitary hormone production (Figure 29.2). Cortisol inhibits ACTH secretion and CRH secretion through negative feedback at both hypothalamic and pituitary levels, a point that is important when considering recovery of the hypothalamic–pituitary–adrenal axis (HPAA) from exogenous glucocorticoid administration. Glucocorticoids with antiinflammatory effects but no effects on the HPAA have not been identified to date. As a result, long-term use of supraphysiological doses may lead to adrenocortical atrophy and decreased adrenal secretory reserve (Chastain et al., 1981; Chastain and Graham, 1979; Hench, 1952; Kemppainen et al., 1982; Moore and Hoenig, 1992). Glucocorticoids inhibit pro-opiomelanocortin (POMC) gene transcription (and therefore ACTH synthesis and secretion) in the anterior pituitary and CRH and vasopressin (AVP) mRNA synthesis and secretion in the hypothalamus. This negative feedback is dependent upon the dose, potency, half-life, and duration of administration of the glucocorticoid administered. Although feedback inhibition is principally mediated by the glucocorticoid receptor (GR), there is evidence of fast-loop feedback, which can account for as much as 50% of the negative feedback effect. Glucocorticoids have shown negative effects on spiking of periventricular nucleus (PVN) neurons in the hypothalamus by an effect blocked by a glucocorticoid receptor antagonist. However, GCs also acutely suppress voltage-activated K+ currents of PVN neurons, and inhibit glutamatergic excitatory postsynaptic currents by PVN neurons within minutes. This effect appears to be due to the release of endocannabinoids, which suppress in a retrograde fashion the presynaptic release of glutamate and γ-amino butyric acid (GABA). Furthermore, GCs induce a rapid facilitatory effect on GABA release by these hypothalamic neurons. There is some evidence that the membrane-bound GRs mediating these rapid effects engage directly or indirectly the Gs-adenylate cyclase-PKA signaling pathway. The combined effect of suppression of excitatory synaptic input with facilitation of inhibition of PVN neurons results in rapid inhibition of PVN output controlling the HPAA (Di et al., 2003; Tasker et al., 2006). The pattern of glucocorticoid presentation influences gene regulation and tissue responses: pulsatility is an important factor for regulation of the HPAA and of tissue responses to glucocorticoids (Russell and Lightman, 2014). In humans, single daily doses of exogenous glucocorticoids are most commonly recommended to be given in the morning to mimic the adrenal gland’s secretory pattern (Tyrrell et al., 1994). Although older reports claimed the existence of a diurnal cortisol variation in dogs and cats, with plasma cortisol level peaking in the morning in dogs and in the evening in cats, carefully designed studies have not confirmed these observations (Kemppainen, 1986). Under basal (nonstressful) conditions, the adrenal gland produces cortisol (hydrocortisone) at about 1 mg/kg body weight daily in most species. In plasma, cortisol is over 90% bound to plasma proteins. The remaining 10% free hormone is the active moiety according to the free-hormone hypothesis. Corticosteroid-binding globulin (CBG), an α2 globulin synthesized by the liver, binds the majority of circulating hormone under normal circumstances. The remainder is free or loosely bound to albumin and is available to exert its effect on target cells. Cortisol is removed from the circulation by the liver, where it is reduced and conjugated to form water-soluble glucuronides and sulfates, which are excreted into the urine (Aron and Tyrrell, 1994; Grote et al., 1993; Hammond, 1990; Tyrrell et al., 1994). Glucocorticoids have four cellular mechanisms of action: (i) the classical genomic mechanism of action caused by the cytosolic glucocorticoid receptor (cGR); (ii) secondary nongenomic effects also initiated by the cGR; (iii) membrane-bound glucocorticoid receptor (mGR)-mediated nongenomic effects; and (iv) nonspecific, nongenomic effects caused by interactions with cellular membranes (Figure 29.3; Loewenberg et al., 2007). Which of these effects is seen will depend upon the tissue and the dose of glucocorticoids (Table 29.2 ) (Stahn et al., 2007; Buttgereit, 2004). Figure 29.3 Genomic and nongenomic cellular mechanisms of glucocorticoid (GC) action. GCs diffuse into cells and bind to the cytoplasmic GR, after which the GC–GR complex translocates into the nucleus. Figure 29.4 Mechanism of transrepression by glucocorticoids and selective glucocorticoid receptor agonists/modulators (SEGRAMs). Simplified overview of the mechanism of action of a classical glucocorticoid versus the mechanism of action of a SEGRAM. GR, glucocorticoid receptor; GRE, glucocorticoid receptor responsive element; TF, transcription factor. Source: https://en.wikipedia.org/wiki/File:SEGRAM_basic_mechanism.tif. Used under CC BY-SA 3.0 http://creativecommons.org/licenses/by-sa/3.0/. Table 29.2 Current knowledge on the relationship between clinical dosing and cellular actions of glucocorticoids. Source: Adapted from Buttgereit et al., 2004. Reproduced with permission of John Wiley & Sons. Replacement or low maintenance Antiinflammatory Initial Antinflammatory (chronic disease) Initial Antiinflammatory (subacute) or chronic immunosuppressive Very severe or life-threatening Immunosuppressive or initial lymphocytolytic cGR, cytosolic glucocorticoid receptor. ?, unknown; −, not relevant; (+), perhaps relevant, but of minor importance; +, relevant; ++, relevant to very relevant; +++, very relevant; ++++, most relevant. Genomic mechanisms mediate most effects associated with eventual protein synthesis, such as low-dose physiological replacement therapy and antiinflammatory and immunomodulatory effects; however, it is now clear that some effects, particularly the more rapid ones seen at high glucocorticoid doses, cannot be explained through this mechanism (see Section Nongenomic Effects of Glucocortoids). The genomic mechanisms of glucocorticoid action are cGR-mediated by the cytoplasmic glucocorticoid receptor. The unliganded cGR is a 94-kD protein that exists in the cytoplasm as a multiprotein complex including heat-shock proteins (Hsp), such as Hsp90, Hsp70, Hsp56, and Hsp40. There is also an interaction with immunophilins, (chaperones such as p23 and Src) and several kinases in the mitogen-activated protein kinase (MAPK) signaling system. The glucocorticoid receptor itself consists of three domains: an N-terminal domain containing transactivation functions, a DNA-binding domain with a “zinc-finger” motif, and a glucocorticoid-binding domain. Binding to the cGR ultimately induces (also known as transactivation) or inhibits (also known as transrepression) the synthesis of regulatory proteins. The genomic effects are generally not observed prior to 30 minutes because of the time necessary for cGR activation/translocation, transcription, and translation. It is estimated that glucocorticoids influence the transcription of ∼1% of the entire genome either directly or indirectly through interaction with transcription factors and coactivators (Stahn et al., 2007). The cholesterol-like structure and low molecular weight (∼300 daltons) allow GCs to pass easily through the cell membrane and bind to the inactive cGR. Conformational changes in the GC/cGR complex result in dissociation from heat shock proteins (HSPs) 70 and 90 followed by migration within 20 minutes to the nucleus. Binding occurs as a homodimer to the glucocorticoid response element (GRE) in association with the activator protein-1 (AP-1) comprised of c-fos and c-jun. Binding of the GC/cGR to a positive GRE (pGRE) results, for example, in induced synthesis of antiinflammatory proteins (e.g., lipocortin 1, IκB, annexin-1, MAP kinase phosphatase 1, and IL-10), as well as metabolic proteins (e.g., those key to gluconeogenesis) often associated with common side effects. Transcription of genes can be inhibited by GCs via direct interaction between the GR and negative GREs (nGRE), such as those associated with pituitary expression and secretion of pro-opiomelanocortin, the precursor to ACTH, which constitutes the negative feedback system in the pituitary (see Figure 29.2s, 29.3) (Loewenberg et al., 2007). Glucocorticoids also suppress expression of inflammatory genes, including interleukin 1 and 2 (IL-1 and IL-2) via the same mechanism (Loewenberg et al., 2007; Stahn et al., 2007). The repressive effect of GR on nuclear factor-κB (NF-κB) regulation is balanced by the NF-κB negative regulation of GR α–mediated transcription, although the mechanism underlying such antagonism is as yet unclear (Duma et al., 2006). Transcription factors may also be displaced from a positive GRE through direct interaction of the factor and the GR. In addition, GR-mediated transcriptional modulation can be achieved through direct protein–protein interaction with proinflammatory transcription factors, such as activator protein-1 (AP-1), NF-κB, or signal transducers and activator of transcription (STAT), leading to inhibition of target gene expression (van der Velden, 1998; Loewenberg et al., 2007). Undesirable effects of GC therapy have generally been ascribed to dimer-mediated transactivation, while beneficial antiinflammatory effects are due to monomer-mediated transrepression of the GR. However, dimer-mediated transactivation is now known to be essential to antiinflammatory activity (Vandevyver et al., 2013). Dissociated GR-ligand agonists (DIGRAs), now preferably called selective glucocorticoid receptor agonist modulators (SEGRAMs, SEGRAs, or SEGRMs), are experimental drugs in development designed to share desirable effects of glucocorticoids with fewer side effects (Schäcke et al., 2007). To the author’s knowledge, none have yet made it to veterinary use. Negative regulation by the GR is associated with inhibition of transcription factor activity (generally proinflammatory) through tethering to such factors. GR homodimer binding to the GRE causes histone acetylation resulting in chromatin remodeling and the association of RNA polymerase II through recruitment of coactivator proteins with acetyltransferase activity, leading to transactivation (e.g., cyclic AMP-responsive element-binding protein). However, when homodimeric GR binds to the negative GRE, either by displacing coactivators or via recruitment of corepressors or histone deacetylases (HDACs), the chromatin structure closes and transcription is inhibited (Schäcke et al., 2007). The GC-induced leucine zipper (GILZ) gene has been identified as a downstream mediator of GC antiinflammatory and immunosuppressive effects, and may eventually be a pharmacological target (Ayroldi et al., 2014). Some of these compounds also have shown reduced tendency for insulin antagonism and therefore metabolic side effects (Brandish et al., 2014). An important mechanism for antiinflammatory and immunosuppressive effects of glucocorticoids is the inhibition of phospholipase A2 (PLA2), the enzyme responsible for the release of arachidonic acid from membranes prior to its further metabolism by the cyclooxygenase (COX) and lipoxygenase (LOX) pathways (Figure 29.5). Glucocorticoids enhance the production of a protein called lipocortin, which inhibits the enzyme phospholipase A2 in the cell membrane, thereby inhibiting the formation of prostaglandins, leukotrienes, and PAF (Figure 29.5). Glucocorticoids also may inhibit other phospholipases, such as phospholipase C (Barragry, 1994; Goldfien, 1992; Sorenson et al., 1988). Lipocortin-1, a 37 kDa member of the annexin superfamily of proteins, plays a major regulatory role in systems as diverse as cell growth regulation and differentiation, neutrophil migration, CNS responses to cytokines, neuroendocrine secretion, and neurodegeneration (Flower and Rothwell, 1994). Figure 29.5 Comparison of the effects of glucocorticoids and nonsteroidal antiinflammatory drugs (NSAIDs) on arachidonic acid–derived mediators of inflammation. Glucocorticoids stimulate the production of lipocortin, which inhibits the activity of plasma phospholipase A2 (PLA2) and thereby inhibits the release of arachidonic acid and indirectly the production of newly formed inflammatory mediators of the cyclooxygenase pathway as well as the lipoxygenase pathway. The net result is a reduction in production of leukotrienes and chemotactic compounds, as well as less formation of thromboxane A2 (TxA2) by thromboxane synthase (TxS), of prostaglandins E2 and F2α by prostaglandin synthase (PGS), and of prostacyclin (PGI2) by prostacyclin synthase (PCS). Glucocorticoid and mineralocorticoid receptors (GR and MR, respectively) form a class of proteins within the nuclear receptor superfamily of receptors including glucocorticoids, mineralocorticoids, estrogens, progesterone, androgen, vitamin D, and retinoic acid. They are widely conserved throughout the species. Glucocorticoid receptors are critical for normal development and differentiation and are essential for life (Duma et al., 2006). For the human GR, there exists a steroid-binding isoform of 777 amino acids, termed α, and a nonsteroid binding β isoform of 742 amino acids, which is identical to the α isoform through the first 727 amino acids (Figure 29.6). Most effects are due to GRα for GR are multiple translation isoforms, and each may undergo different types and degrees of posttranslational modification. Initially, it was thought that GRβ was not physiologically significant but it is now known that it is constitutively expressed in the nucleus and antagonizes the activity of GRα (Ayroldi et al., 2014). GRα is considered the classical GR responsible for most genomic actions, whereas the β isoform has been implicated in the conveyance of glucocorticoid resistance, and possibly a role in autoimmune and inflammatory disorders. It has been suggested that GRβ inhibits GRα transcriptional activity by interference with formation of the coactivator complex. Figure 29.6 Schematic structure of the human genes encoding the glucocorticoid receptor (GR) and mineralocorticoid receptor (MR). Splice variants have been described for both receptors; in the case of the glucocorticoid receptor, there is evidence that the GR β isoform can act as a dominant negative inhibitor of GR α action. mRNA, messenger ribonucleic acid. Source: Stewart, 2003. Reproduced with permission of Elsevier. Much remains to be clarified mechanistically, but it has been suggested that GRβ overexpression may either be neutral, or might lead to anti- or proinflammatory states. There would be pharmacological relevance of GRβ overexpression as an antiinflammatory or to suppress metabolic side effects of glucocorticoids. To date, most studies report higher expression of GRβ in autoimmune, inflammatory, or glucocorticoid-resistant states. Neutrophils have a normally high β : α GR ratio, and this may account for their relative glucocorticoid-resistance. However, no study has yet evaluated the effect of acute or chronic glucocorticoid administration on GRβ expression. Nonetheless, proinflammatory cytokines induce an overexpression of GRβ and expression of this GR isoform has been postulated as a possible predictor of therapeutic failure with glucocorticoid therapy (Duma et al., 2006). The function of GRs can be subsequently altered by posttranslational modifications, such as phosphorylation, ubiquitination, and SUMOylation. Ultimately, such changes may alter subcellular distribution, transcriptional activity, and protein–protein interactions. Ubiquitination is believed to be a major mechanism by which the degradation rates of GRs are regulated, regulating targeting to proteasome degradation. This is a highly conserved mechanism among eukaryotes. Addition of a small ubiquitin-related modifier-1 (SUMO-1) is called SUMOylation and may also impact protein stability and localization. For example, of significance to immunosuppressive or anticancer therapy, the phosphorylation state of the GR may impact glucocorticoid sensitivity during the cell cycle. Glucocorticoid treatment increases phosphorylation during the S phase, but not during the G2 or M phases. As a result, cells synchronized to the S phase were responsive to glucocorticoids, whereas those in G2/M phase were not (Duma et al., 2006). In shock and pulse immunosuppressive therapy, very high doses of glucocorticoids are administered. At such doses, cGRs are believed to be saturated (Table 29.2 ) and effects are unlikely to be limited to receptor-mediated phenomena. At high GC concentrations, nonspecific effects due to direct interaction with the cell membrane lipid may occur (Figure 29.3). These effects are too rapid to be regulated transcriptionally and are termed nongenomic but represent several possible mechanisms. Rapid GC effects might occur by interaction of GCs with specific membrane receptors. For example, a plasma-membrane form of GR has been shown in B cells and peripheral blood mononuclear cells. GCs may also act on the plasma membrane by nonspecific physicochemical mechanisms, particularly when at high concentrations. Through such mechanisms, they may inhibit Na+ and Ca++ plasma membrane transport or increase H+ leak of the mitochondria. The former mechanism in lymphocytes has been implicated as part of the rapid immunosuppression induced by glucocorticoids. The effects of GCs on ion fluxes and vascular permeability are independent of protein synthesis (Muller and Rankawitz, 1991; Reul et al., 1990). In fact, the rapid effect of GCs at inhibition of allergic conditions has been shown to be caused by a reduction in intracellular calcium (Zhou et al., 2008). Nongenomic effects of glucocorticoids have also been described on different cellular processes, such as actin polymerization, neuronal membrane conductance, and other signal transduction mechanisms. For example, short-term glucocorticoid treatment reduced antigen-induced phosphorylation of mitogen-activated protein (MAP)–extracellular signal-regulated kinase (ERK, MEK), as well as reduced phospholipase A2 (PLA2) (Song and Buttgereit, 2006). Specific membrane-associated receptors for glucocorticoids may be involved in the rapid effects of these agents in shock conditions (Gametchu et al., 1991; Grote et al., 1993; Liposits and Bohn, 1993). Glucocorticoids as cholesterol-like compounds, may change membrane fluidity, thereby regulating plasma membrane GABAA, EGF, insulin-like growth factor-I (IGF-I), and dopamine receptors. Receptor-mediated insertion of steroid hormones into DNA may also take place, with the steroid acting as a transcription factor. These diverse modes of action provide for integrated rapid and/or prolonged effects to address the physiological needs of the individual (Brann et al., 1995). Glucocorticoids can induce the rapid phosphorylation and membrane translocation of annexin-1, an important antiinflammatory protein. They also have been shown to activate endothelial nitric oxide synthase (eNOS) via actions on phosphatidylinositol 3-kinase and protein kinase B, resulting in vasodilatation associated with cardio protective effects of glucocorticoids. Neuroprotection following high-dose glucocorticoids may also be associated with a nongenomic effect, again possibly by eNOS activation and increased cerebral blood flow (Song and Buttgereit, 2006). Membrane-bound states of the cytosolic glucocorticoid and mineralocorticoid receptors have also been described. Aldosterone receptors on plasma membranes of lymphocytes and smooth muscle cells appear to trigger changes in Ca++, IP3, cAMP, and protein kinase C. Supporting clinical significance, the number of plasma membrane-bound GRs correlates with the ability of GCs to induce lymphocyte death. Further work is necessary to clarify the functional role and cellular mechanisms associated with membrane-bound GR. Current thinking is that there is only one cytosolic GR, which may be either unliganded and membrane-associated, or liganded and act as a “classical” nuclear transcription factor. In future pharmaceutical development, glucocorticoids with preference for membrane-bound GRs may have enhanced immunosuppressive activity with fewer side effects (Buttgereit et al., 2004; Song and Buttgereit, 2006; Loewenberg et al., 2007). A principal role of glucocorticoids is the maintenance of fluid homeostasis by regulation of volume and composition of body fluids and by being permissive for essential cellular metabolism. Without glucocorticoids, an animal cannot survive a stressful incident. The glucocorticoids have widespread effects because they influence the function of most cells in the body. Glucocorticoids in physiological quantities are essential for the dilution of the renal filtrate into the hyposthenuric range. Other physiological roles of glucocorticoids are to increase gluconeogenesis, decrease protein synthesis, and increase lipolysis with the release of glycerol and free fatty acids (an insulin-antagonistic effect). Although many of the effects are dose related, glucocorticoids may also act in a permissive manner to optimize certain cellular reactions such as the gluconeogenesis stimulated by glucagon and catecholamines. The physiological effects of glucocorticoids in the fed state are not very significant; however, during fasting, glucocorticoids contribute to the maintenance of glucose concentrations by increasing the release of glucose by the liver and increasing gluconeogenesis and glycogen deposition by stimulating glycogen synthase. The effect on muscle is catabolic because glucose uptake decreases and amino acid release (gluconeogenesis) increases. Glucocorticoids stimulate adipocyte differentiation, promoting adipogenesis through activation of key adipocyte differentiation genes such as those coding for lipoprotein lipase, glycerol-3-phosphate dehydrogenase, and leptin. Glucocorticoids also are permissive for activity of the hormone-sensitive lipase (HSL), which is responsible for mobilization of free fatty acids from adipose stores. Lipolysis is stimulated, therefore, when insulin is lacking or being greatly antagonized; ketogenesis may result (Aron and Tyrrell, 1994; Ferguson, 1985a; Goldfien, 1992; Haynes, 1990; Melby, 1974; Wilcke and Davis, 1982). Glucocorticoids also maintain microcirculation, normal vascular permeability, and stability of lysosomal membranes, and suppress inflammatory reactions, although these functions are more commonly associated with the pharmacological effects of glucocorticoids (Aron and Tyrrell, 1994; Tyrrell et al., 1994). Glucocorticoids play a physiological role in the development of pulmonary surfactant in the near-term fetus, allowing an adaptation to air breathing. GCs stimulate lung maturation through the synthesis of surfactant proteins. GR knockout mice do not survive because of lung atelectasis. Prematurity with delayed development of the adrenal axis in foals has been suspected as a cause of neonatal respiratory distress syndrome. Although glucocorticoids stimulate growth hormone (GH) gene transcription in physiological quantities, glucocorticoids in excess inhibit linear skeletal growth, via catabolic effects on connective tissue and muscle and inhibition of IFG-1 effects. GCs also stimulate the adrenal medullary enzyme converting norepinephrine to epinephrine (Aron and Tyrrell, 1994; Tyrrell et al., 1994; Stewart, 2003). Glucocorticoids have an antagonistic effect to that of insulin, leading to increased glucose production from amino acids (gluconeogenesis) and reduced incorporation of amino acids into protein. As stated above, glucocorticoids enhance lipolysis; however, glucocorticoid excess (pharmacological amounts) or spontaneous hyperadrenocorticism may result in redistribution of fat because glucocorticoids stimulate appetite, thereby stimulating hyperinsulinemia, which results in lipogenesis. As a result, diabetes mellitus may result from prolonged glucocorticoid use at high dosages in animals with diminished insulin secretory capacity (prediabetics). Muscle wasting and weakness are not uncommon with glucocorticoid excess; although glucocorticoids stimulate protein and RNA synthesis in the liver, they have catabolic effects in lymphoid and connective tissue, muscle, fat, and skin. While not usually a recognizable clinical problem in domestic animals, osteoporosis may result in people with Cushing’s syndrome or on chronic glucocorticoid administration. The problem likely is most significant in areas of healing bone; glucocorticoids directly inhibit bone formation by inhibiting osteoblast proliferation and the synthesis of bone matrix while stimulating osteoclast activity. In addition, glucocorticoids potentiate the action of parathyroid hormone (PTH) and 1,24-dihydroxycholecalciferol (1,25(OH)2-D3) and inhibit the gut absorption of calcium, an effect that can be used to advantage in hypercalcemic states. However, in the young animal, the catabolic effects of excessive amounts of glucocorticoid reduce growth. In children, this reduced growth is not prevented by growth hormone (Aron and Tyrrell, 1994; Tyrrell et al., 1994). In adult dogs, 2 mg/kg prednisone for 30 days reduced bone mineral density by 14% as measured by helical computer tomography (Costa et al., 2010). Glucocorticoid use invariably leads to polyuria and polydipsia via inhibition of ADH release and action, as well as alteration of the animal’s psyche, resulting in increased water intake. No glucocorticoid given in large doses is completely devoid of mineralocorticoid (salt-retaining and K+-losing) activity; therefore, excessive use may precipitate or exacerbate hypertension and induce hypokalemia. In part by increasing extracellular fluid volume, glucocorticoids increase the glomerular filtration rate and are required in physiological amounts for maximal dilution of urine (Ferguson, 1985a; Melby, 1974; Nakamoto et al., 1992; Smets et al., 2012). Often the desired result of a therapeutic application, antiinflammatory effects of glucocorticoids are primarily seen at pharmacological doses. Glucocorticoids result in alterations in the concentration, distribution, and function of peripheral leukocytes and in inhibition of phospholipase A2 activity in the plasma membranes of these cells. Glucocorticoids act indirectly by inducing lipocortin synthesis, which in turn inhibits arachidonic acid release from membrane-bound stores, and also by inducing transforming growth factor-β (TGF-β) expression, which subsequently blocks cytokine synthesis and T-cell activation. In addition to contributing to maintenance of the microcirculation and cell membrane integrity, glucocorticoids interfere with progressive dissolution and disruption of connective tissue and cells, possibly by stabilizing lysosomal membranes (Aron and Tyrrell, 1994; Aucoin, 1982; Barragry, 1994). Although lysosomal stabilization by glucocorticoids has been demonstrated experimentally, it is hard to know what benefit these effects have in clinical situations. Glucocorticoids also decrease formation of induced histamine (histamine produced locally by cells during injury), the action of which is not effectively blocked by standard doses of antihistamines. They also antagonize toxins and kinins, reducing the resultant inflammation. It is important to realize, however, that most of their effects are nonspecific; that is, they have profound metabolic effects regardless of the initial insult. Glucocorticoids are used to advantage to suppress both the number of cells and the actions of the immune system. The suppressive effects on cell-mediated immunity predominate over those on humoral immunity. Antibody production is generally unaffected by moderate dosages of glucocorticoids and is inhibited only at high dosages and with long-term therapy. They cause lymphopenia and eosinopenia, an effect secondary to cell redistribution and/or lysis, and lead to increased vascular demargination of neutrophils from the vascular bed to lymphoid tissue. Glucocorticoids inhibit virus-induced interferon synthesis and diminish the functional capacity of monocytes, macrophages, and eosinophils through inhibition of the formation of ILs such as IL-1 (macrophages), IL-2 (lymphocytes), IL-3, and IL-6 and other chemotactic factors. Glucocorticoids can induce apoptosis on normal lymphoid cells and play a key role in the physiology of thymic selection. They inhibit monocyte differentiation into macrophages and macrophage phagocytosis and cytotoxic activity (Barragry, 1994; Ehrich et al., 1992; McDonald and Langston, 1994; Melby, 1974; Tyrrell et al., 1994; Buttgereit et al., 2004). In recent years, greater understanding of the mechanisms of GC inhibition of cell-mediated immunity has developed, and the GC-induced leucine zipper (GILZ) gene has emerged as a critical mediator. In response to GCs, GILZ up-regulates and inhibits T cells through inhibition of anti-CD3-induced activation and apoptosis. GILZ also interacts with and inhibits NF-κB. Other GILZ targets have been identified, including AP-1, Raf-1, and Ras, and each has been implicated in the effects of GCs. GILZ silencing diminishes the antiproliferative activity of dexamethasone and reduces inhibition of cytokine-induced COX-2 expression (Ayroldi and Riccardi, 2009; Ayroldi et al., 2014). In addition to the GILZ-mediated effects, GCs appear to up-regulate repair-phase cytokines like TGF-β and platelet-derived growth factor (PDGF), possibly explaining the suppressive effect on healing and fibrosis (Pallardy and Biola, 1998; Buttgereit et al., 2004). In the clinical setting, GCs are used for their ability to induce apoptosis of malignant lymphoid cells. The mechanisms of apoptosis induced by glucocorticoids fall roughly in two categories, depending on the type of lymphocytes: induction of “death genes” such as IκB and c-jun or repression of survival factors such as AP-1 and c-myc. By inhibiting the production of Th1 cytokines, glucocorticoids may enhance Th2 cell activity and generate a long-lasting state of tolerance. In addition to indirect effects on electrolyte metabolism, glucocorticoids have direct positive chronotropic and inotropic actions on the heart. They appear to block the increased permeability of capillaries induced by acute inflammation, reducing transport of protein into damaged areas and maintaining microcirculation. Because glucocorticoids are necessary for maximal catecholamine sensitivity, they contribute to maintenance of vascular tone (Ferguson et al., 1978; Nakamoto et al., 1992). In shock, production of vasoactive products of lipid peroxidation (arachidonic acid cascade), such as the vasoconstrictor thromboxane A2, may be decreased by glucocorticoids, but probably only in the early stage of cell disruption. Glucocorticoids cause vasoconstriction when applied directly to vessels. They decrease capillary permeability by inhibiting the activity of kinins and bacterial endotoxins and by reducing the amount of histamine released by basophils. Glucocorticoids may induce hypertension in animals and humans through the following mechanisms: (i) activation of the renin–angiotensin system due to an increase in plasma renin substrate; (ii) reduced activity of the hypotensive kallikrein–kinin system, prostaglandins (PGs), and the endothelium-derived relaxing factor, nitric oxide (NO); and (iii) increased pressor responses to angiotensin II and norepinephrine. Furthermore, the number of angiotensin II type 1 receptors of vascular smooth muscle cells is significantly increased by glucocorticoids (Saruta, 1996). Glucocorticoids increase the number and affinity of β-adrenergic receptors. Glucocorticoids prevent receptor down-regulation and therefore tachyphylaxis, resulting in potentiation of the effects of β-adrenergic agonists on bronchial smooth muscle, an important effect in the asthmatic patient (Sprung et al., 1984; Tyrrell et al., 1994; Wilcke and Davis, 1982). Although rarely described in domestic animals, glucocorticoids (or lack of them) have marked effects on the psyche, resulting in a form of mental, as well as physical, dependence (Ferguson, 1985a; Metz et al., 1982). It is known that pretreatment of neonatal rats with dexamethasone provides protection against hypoxic–ischemic brain damage. This effect is likely mediated via glucocorticoid receptors, because glucocorticoid receptor antagonist RU38486 reverses the benefit. The neuroprotection also appears to be related to alterations in cerebral metabolism. Glucose utilization is reduced prior to hypoxia–ischemia and is better maintained following dexamethasone. High-energy phosphates in the brain are higher in dexamethasone-treated animals. Thus, glucocorticoids may provide their protection against hypoxic–ischemic damage by decreasing basal metabolic energy requirements and/or increasing the availability or efficiency of use of energy substrates (Tuor, 1997). Glucocorticoids, in addition to being diabetogenic, also have marked effects on hypothalamic and pituitary function. ACTH, β-lipotropin, thyroid-stimulating hormone (TSH), follicle-stimulating hormone (FSH), and growth hormone (GH) synthesis and secretion are all suppressed; however, β-endorphin levels are unaffected. Glucocorticoids, even at “physiological” dosages (0.22 mg/kg prednisolone once daily orally in the dog), result in HPAA suppression, as indicated by reduction in the ACTH-stimulated cortisol concentration increment and by reduction of the ratio of the zona fasciculata and reticularis to zona glomerulosa in the adrenal gland. Antiinflammatory dosages of prednisolone (0.5 mg/kg q 12 h orally) resulted in adrenal suppression within 2 weeks of therapy (Chastain and Graham, 1979). In another study in dogs, 1 month of the same dosage of prednisolone orally resulted in profound suppression of endogenous plasma ACTH and cortisol concentrations, CRH-stimulated ACTH release, and ACTH-stimulated cortisol release. However, following withdrawal of the prednisolone, the HPAA returned to normal within 2 weeks (Moore and Hoenig, 1992). A similar ability to recover from exogenous glucocorticoids was seen in the cat. A dosage of 2 mg/kg q 12 h of methylprednisolone given orally for 7 days resulted in suppression of ACTH-stimulated cortisol and CRH-stimulated ACTH, but these changes completely reversed by 7 days after withdrawal of the exogenous glucocorticoid (Crager et al., 1994). Higher dosages and long-acting preparations (dexamethasone, triamcinolone, depot products) may result in more pronounced HPAA suppression (Kemppainen and Sartin, 1984; Kemppainen, 1986; Kemppainen et al., 1982). Even hydrocortisone at 10 mg/kg twice a day for 4 months led to ultrasonographically evident adrenal atrophy that was reversible after 1 month of withdrawal (Pey et al., 2012). The effect of glucocorticoids on glucose metabolism is time and dose dependent; insulin and glucose concentrations, or glucose tolerance, were not significantly altered by the administration for 28 days of an antiinflammatory dosage of oral prednisone (Moore and Hoenig, 1993). However, daily administration of high dosages of dexamethasone and growth hormone is a reliable model for induction of diabetes mellitus in the cat (Hoenig et al., 2000). There also have been case reports of diabetes mellitus induced in dogs after administration of corticosteroids and methylprednisolone pulse therapy (Jeffers et al., 1991). Pharmacological doses of glucocorticoids generally reduce serum thyroid hormone concentrations, presumably through suppression of pituitary TSH. These effects have been well documented in the dog, are not as significant in the cat, and are not well studied in other domestic species (Ferguson and Peterson, 1992; Kaptein et al., 1992; Moore et al., 1993). The metabolic consequences of these lowered concentrations of thyroid hormones are not known in the dog; however, a state of hypothyroidism is not believed to be the result (Jennings and Ferguson, 1984). Physiological glucocorticoid responses contribute to the maintenance of the gastric mucosal integrity following ulcerogenic stimuli. The role of glucocorticoids in gastroprotection becomes especially important where there is deficiency of PGs or NO or desensitization of intestinal sensory neurons (CSN). In rats with normal corticosterone, these insults do not result in gastric mucosal disruption, but do in adrenalectomized rats (Filaretova et al., 2007). These findings may explain the observation of bloody diarrhea in dogs with glucocorticoid deficiency and/or Addison’s disease. However, pharmacological doses of glucocorticoids stimulate excessive production of acid and pepsin in the stomach and may cause peptic ulcer. In an experimental canine fundic pouch model of acid secretion, the effect of prednisolone (2 mg/kg per day given parenterally for three doses, 2 weeks or 12 weeks) on gastric mucosal H+ permeability was studied. Acute administration (<2 weeks) had no effect. Chronic administration (12 weeks) increased slightly the basal H+ secretion rate, and increased mucosal permeability by 50% when simultaneously administered with aspirin (Chung et al., 1978). Similarly, 0.25 mg/kg SC q 12 h for 3 days induced endoscopically evident gastric lesions in dogs (Boston et al., 2003). Glucocorticoid lesions are exacerbated by simultaneous administration of nonsteroidal antiinflammatory drugs (NSAIDs) (Dow et al., 1990; Narita et al., 2007). In rats, dexamethasone-induced ulceration has led to alterations in the phospholipid fatty acid profile (increased linoleic acid and decrease in other polyunsaturated fatty acids, PUFAs). PUFA supplementation or the administration of an H2 receptor antagonist reduced ulcerization with near normalization of changes in the phospholipid fatty acid profile (Manjari and Das, 2000). Prednisolone and dexamethasone, studied in canine colon cell culture, had no effect on the intestinal cell tight junction barrier function, but did prevent the increase in permeability induced by TNF-α. Specifically, glucocorticoids, acting through nuclear receptors, inhibited the TNF-α–induced increase in myosin light chain kinase protein expression, which was shown to mediate increased intestinal tight junction permeability (Boivin et al., 2007). Glucocorticoids facilitate fat absorption and appear to antagonize the effect of vitamin D on calcium absorption. Therefore, glucocorticoids are employed in chronic hypercalcemic states in an attempt to inhibit gastrointestinal calcium absorption (Aron and Tyrrell, 1994; Tyrrell et al., 1994). Glucocorticoid therapy may cause calcinosis cutis in dogs, but no specific dose or set of factors has been identified to consistently cause it (Doerr et al., 2013). Although the natural corticosteroids can be obtained from animal adrenal glands, they are usually synthesized from cholic acid or steroid sapogenins found in plants of the Liliaceae and Dioscoreaceae families. Further modifications of these steroids have led to the marketing of a large group of synthetic steroids with special characteristics that are pharmacologically and therapeutically important (Table 29.3 and Figures 29.7 and 29.8). Table 29.3 Characteristics of various glucocorticoid bases Effective antiinflammatory time equals the HPAA suppression time in most cases. However, the therapeutic success and fewer side effects with alternate-day therapy stem from the fact that some preparations have slightly longer antiinflammatory or immunosuppressive action than their action to suppress the HPAA. aCompared with hydrocortisone on a mg-for-mg basis. HPAA, hypothalamic–pituitary–adrenal axis. Figure 29.7 Structure–activity relationships of glucocorticoids. Shown on the structure of the compound cortisol are the important structural sites determining the activity of a glucocorticoid base. A: 3 keto group essential for glucocorticoid activity; B: 4,5 double bond essential for glucocorticoid activity; C: 11 hydroxyl essential for optimal glucocorticoid activity; D: 17 α hydroxyl is important for glucocorticoid activity; E: 16 methylation or fluorination reduces mineralocorticoid activity considerably and increases glucocorticoid activity; F: 20 keto group is important for glucocorticoid activity; G: 21 hydroxyl is essential for mineralocorticoid activity and is the site of esterification. Figure 29.8 Structures of common glucocorticoids used in veterinary medicine. The key elements of structure differing from cortisol are identified by the outlined areas.
Glucocorticoids, Mineralocorticoids, and Adrenolytic Drugs
Glucocorticoids
Management Of Hypoadrenocorticism
Management of Nonadrenal Disorders: Inflammatory, Allergic, and Autoimmune Disorders
Review Of Physiology
Biosynthesis of Steroids
Enzyme name
Gene
Cholesterol side-chain cleavage (SCC) (desmolase)
CYP11A1
3β-hydroxysteroid dehydrogenase (3β-HSD) (type II isozyme)
HSD3B2
17α-hydroxylase/17,20 lyase
CYP17
21-Hydroxylase
CYP21A2
11β-Hydroxylase
CYP11B1
Aldosterone synthase
CYP11B2
Hypothalamic–Pituitary–Adrenal Axis
Cellular mechanisms of negative feedback:
Therapeutic significance:
Plasma Binding, Metabolism, and Excretion
Molecular Mechanisms of Action
Left side of figure: (a) Ligated GR directly inhibits proinflammatory transcription factors such as AP-1,NF-κB and STAT (b) Ligated GR actively suppresses transcription (transrepression) of inflammatory genes (such as IL-1 and IL-2) through binding to negative GREs (nGREs). GC-induced transrepression is slow because time is required before RNA and protein levels of target genes are fully degraded. (c) Activated GR induces transcription (transactivation) of immunosuppressive genes (e.g., lipocortin-1) via positive GREs (pGREs). Transactivation of genes that encode regulator proteins is less slow (medium slow) compared to transrepression.
Right side of figure: GCs induce rapid effects (occurring within minutes) on transmembrane currents, signal transduction (such as MAPK signaling pathways), second-messenger cascades, or intracellular Ca++ mobilization. Nongenomic GC effects are mediated by cytosolic or membrane-bound GRs, or via nonspecific interactions with cell membranes.
In this simplified scheme, no GR–chaperones are depicted.
cGR, cytosolic glucocorticoid receptor; GC, glucocorticoid; GRE, glucocorticoid receptor responsive element; MAPK, mitogen activated protein kinase; mGR, membrane-bound glucocorticoid receptor; TCR, T-cell receptor; TF, transcription factor. Source: Loewenberg et al., 2007. Reproduced with permission of Elsevier.
Daily prednisolonedosage (mg/kg)
Therapeutic application
Genomic actions (% receptor saturation)
Nongenomic nonspecific
Nongenomic cGR-mediated
Low (≤0.2)
+ (<50%)
−
?
Medium (0.2–0.5)
++ (50–100%)
(+)
(+)
High (0.5–1)
+++ (almost 100%)
+
+
Very high (1–3)
Acute antiinflammatory or life-threatening immunosuppressive
+++ (almost 100%)
+++
++
Pulse or shock therapy (≥3)
++++ (100%)
++++
+++
Genomic Mechanisms of Action
Genomic effects via the cytosolic glucocorticoid receptor (cGR):
Selective glucocorticoid receptor modulators (SEGRMs) and agonists (SEGRAs):
Mechanism of action (Figures 29.4):
Induction of lipocortin and inhibition of phospholipase A2:
Steroid receptor isoforms:
Posttranslational modifications:
Nongenomic Effects of Glucocorticoids
Physiological Effects of Glucocorticoids
Pharmacological Effects
Growth and Development
Energy Metabolism
Water and Electrolyte Balance
Immune and Hematological Effects
Cardiorespiratory Effects
CNS Effects
Endocrine Effects
Gastrointestinal Effects
Chemistry
Source
Potency
Drug
Glucocorticoida
Mineralocorticoid
HPAA suppression
Alternate-day therapy possible?
Short-acting (duration of action: <24 hours)
Hydrocortisone
1
++
+
No (too short)
Cortisone
0.8
++
+
Yes (not ideal)
Prednisone
4
+
+
Yes
Prednisolone
4
+
+
Yes
Methylprednisolone
5
+
+
Yes
Intermediate-acting (duration of action: 24–48 hours)
Triamcinolone
5
0
++
No
Long-acting (duration of action: <48 hours)
Flumethasone
15
0
+++
No
Dexamethasone
30
0
+++
No
Betamethasone
30
0
+++
No
Structure–Activity Relationships
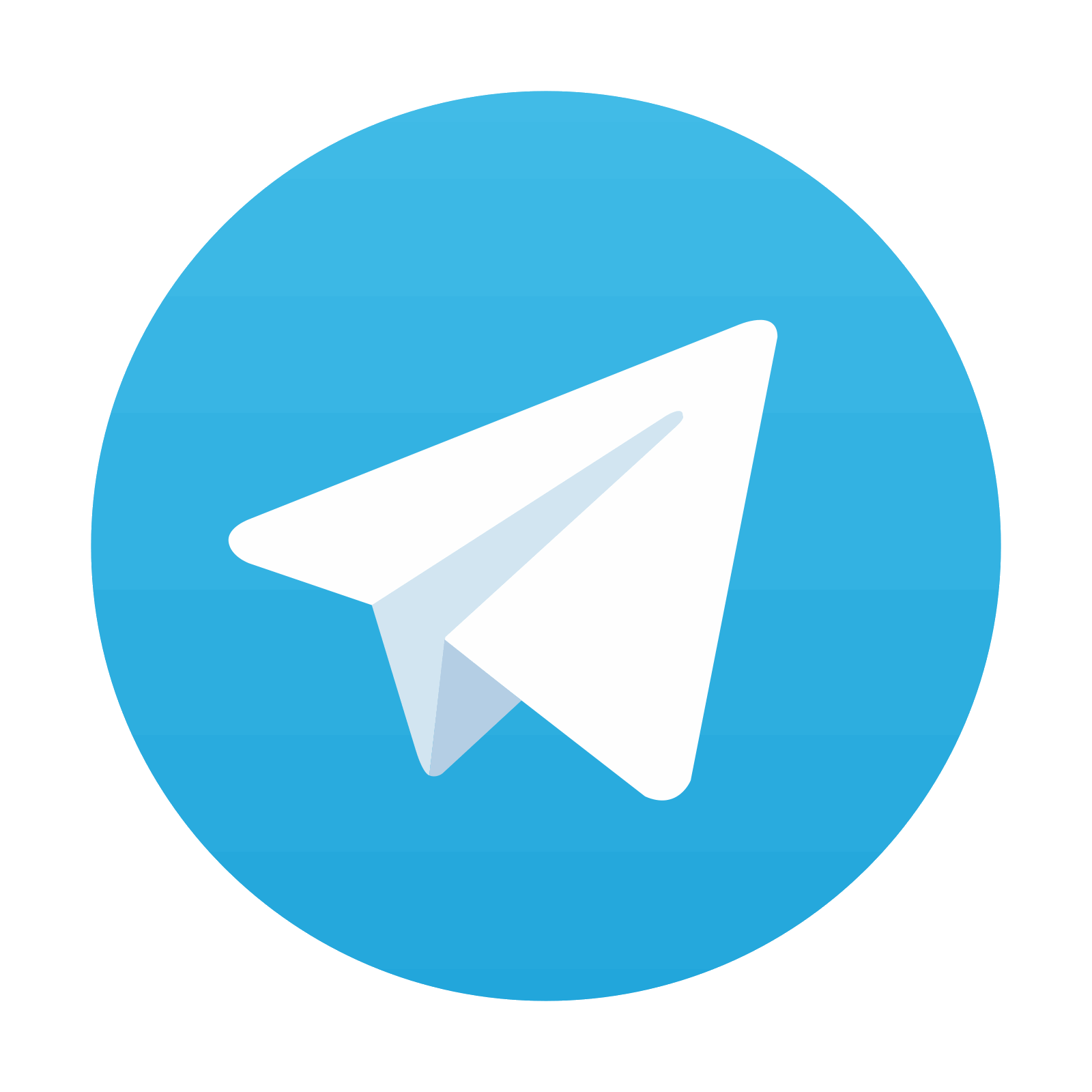
Stay updated, free articles. Join our Telegram channel
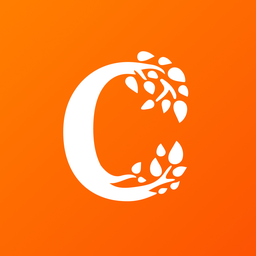
Full access? Get Clinical Tree
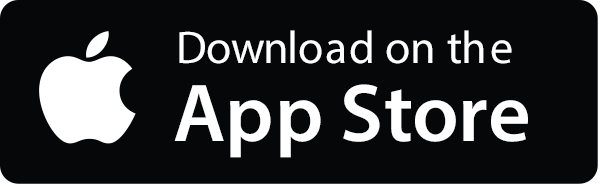
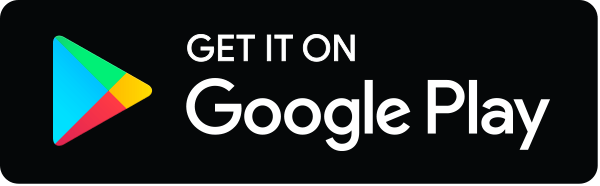